INTRODUCTION
There are several forms of dietary treatment for epilepsy; arguably, the most important and effective is the ketogenic diet (KD). It is a high-fat, low-carbohydrate, and adequate-protein regimen. The ketogenic diet is effective and safe, but, like any medical treatment for epilepsy, it must be judiciously applied and carefully monitored to achieve the best results.
HISTORY
There are biblical references to the salutary effects of starvation upon seizure control, but the earliest scientific reports emerged in the 1920s. Geyelin at the Presbyterian Hospital carefully studied the beneficial effects of starvation upon seizures (1). Shortly thereafter, Wilder proposed a high-fat diet to mimic these effects (2). Under the mistaken notion that ketone bodies were simply toxic and sedating metabolic waste products of fatty acid degradation, the diet was originally viewed as similar to other treatments, including bromides and phenobarbital.
In the 1950s, however, it was discovered that a separate pathway synthesized the ketone bodies, acetoacetate, and 3-hydroxybutyrate; and in 1961 Krebs suggested that ketone bodies were fuels for respiration. In 1967, Owen et al proved that ketone bodies were the major fuel for brain metabolism during starvation (3). Appleton and De Vivo later showed in experimental animals that the utilization of ketone bodies during starvation altered brain metabolites and increased cerebral energy reserves (4). Huttenlocher showed that the level of ketosis correlated with efficacy (5).
There are several different variations of the ketogenic diet, but the mostly widely used regimen uses long-chain triglycerides (LCT) in the form of heavy cream, butter, and meat fat. We will review the scientific basis, effectiveness, and safety of the LCT diet and then briefly review other forms of dietary therapy.
Scientific Basis of the Diet
Like many potent antiseizure treatments, the ketogenic diet functions through multiple diverse and complementary mechanisms (6). This provides a broad spectrum of action. Some knowledge of the mechanisms may aid the clinician in better understanding the indications and contraindications, and also provide a basis for rationally selecting complementary treatments. An in-depth exploration of each of these mechanism is beyond the scope of this chapter, but the interested reader is referred to a recent topical review (6).
Improved Cerebral Energy Reserves and Increased GABA Synthesis
Ketone bodies derive from the metabolism of nonesterified fatty acids. During the fasting state, the fall in blood glucose reduces plasma insulin production, stimulates lipolysis in fatty tissues, and increases the flux of nonesterified fatty acids to the liver. Nonesterified fatty acids can be esterified or metabolized to ketone bodies. The fate of fatty acids in the liver is determined, at least in part, by the carbohydrate status of the host (7). A critical component of this regulation is malonyl-CoA, an intermediate in the pathway of lipogenesis (8,9). Malonyl-CoA inhibits carnitine acyltransferase I, which is needed to shuttle long chain fatty acyl-CoA into the mitochondria for oxidation. The production of glucose from glycogen provides the carbon source for lipogenesis and, in particular, malonyl-CoA. If glucose is reduced, so is malonyl-CoA. The reduction in malonyl-CoA decreases the inhibition upon (or increases the net activity of) carnitine acyltransferase. This allows more movement of fatty acids into the mitochondria, where fatty acyl-CoA is converted to acetyl-CoA, and later acetoacetate. Acetoacetate is in equilibrium with beta-hydroxybutyrate, the major ketone body utilized by the brain.
Passage of ketone bodies into the brain may be the critical factor limiting the rate of brain utilization of ketone bodies. Movement of ketone bodies into the brain relies upon the monocarboxylic transport system, MCT-1. This is up-regulated during fasting in adults and during milk feeding in neonates (10,11). Fasting studies in humans demonstrated that the brain’s ability to extract ketone bodies is inversely related to the age of the subject (3). In contrast to glucose, ketone bodies can pass directly into mitochondria without being processed in the cytosol. Also, in contrast to glucose, ketone bodies may be used directly by neurons for metabolism (12,13).
Once inside the mitochondria, beta-hydroxybutyrate is converted to acetoacetate (AcAc), and then to acetoacetyl-CoA (AcAC-CoA). The enzyme that facilitates this is 3-oxoacid-CoA transferase or succinyl-CoA-acetoacetate-CoA transferase. As the name implies, this conversion requires commensurate conversion of succinyl-CoA to succinate. It is possible that reduced blood glucose and increased blood ketone may be needed to induce the activity of this enzyme (14).
Scientific studies of the ketogenic diet have revealed important biochemical and metabolic observations. Appleton and De Vivo developed an animal model to permit study of the effect of the KD on cerebral metabolism (Figure 70.1) (4). Adult male albino rats were placed on either a high-fat diet containing (by weight) 38% corn oil, 38% lard, 11% vitamin-free casein, 6.8% glucose, 4% U.S.P. salt mixture, and 2.2% vitamin diet fortification mixture or a high-carbohydrate diet containing (by weight) 50% glucose, 28.8% vitamin-free casein, 7.5% corn oil, 7.5% lard, 4% U.S.P. salt mixture, and 2.2% vitamin diet fortification mixture. Parallel studies were conducted to evaluate electroconvulsive shock responses and biochemical alterations. These studies revealed that the mean voltage necessary to produce a minimal convulsion remained constant for 12 days before the high-fat diet was started and for about 10 days after beginning the feedings (69.75 ± 1.88 volts). After 10 to 12 days on the high-fat diet, the intensity of the convulsive response to the established voltage decreased, necessitating an increase in voltage to re-establish a minimal convulsive response. Approximately 20 days after beginning the high-fat diet, a new convulsive threshold was achieved (81.25 ± 2.39 volts) (P < .01). When the high-fat diet was replaced by the high-carbohydrate diet, a rapid change in response to the voltage was observed. Within 48 hours the animal exhibited a maximal convulsion to the electrical stimulus, which had previously produced only a minimal convulsion, and the mean voltage to produce a minimal convulsion returned to the pre-study value (70.75 ± 1.37 volts).
FIGURE 70.1 The effect in adult rats of dietary manipulation on the electroconvulsive threshold.
Source: Abstracted from Ref. (4). Appleton DB, De Vivo DC. An animal model for the ketogenic diet. Epilepsia. 1974;15(2): 211–227. With permission from John Wiley & Sons.
Blood concentrations of beta-hydroxybutyrate, acetoacetate, chloride, esterified fatty acids, triglycerides, cholesterol and total lipids increased in the rats on the high-fat diet. Brain levels of beta-hydroxybutyrate and sodium were also significantly increased in the fat-fed rats.
De Vivo et al reported the change in cerebral metabolites in chronically ketotic rats, and found no changes in brain water content, electrolytes, and pH (15). As expected, fat-fed rats had significantly lower blood glucose concentrations and higher blood beta-hydroxybutyrate and acetoacetate concentrations. More importantly, brain concentrations of ATP, glycogen, glucose-6-phosphate, pyruvate, lactate, beta-hydroxybutyrate, citrate, alpha-ketoglutarate, and alanine were higher and the brain concentrations of fructose 1,6-diphosphate, aspartate, ADP, creatine, cyclic nucleotides, acid-insoluble CoA, and total CoA were lower in the fat-fed group.
The biochemical implications of these results can be grouped into three major categories:
1. Glycolysis and glucose flux are reduced during ketosis. As Wilder originally postulated, the effects of eating a high-fat, low-carbohydrate diet are similar to the biochemical consequences of starvation (2). In this regard, the reduction in glycolysis makes physiologic sense, since the starving organism would want to make every attempt to preserve the rather meager stores of glycogen, and to provide the brain with an alternate, more substantial fuel for metabolism (ketone bodies from fats). Measurements of glucose flux in children and adults during ketosis have confirmed the reduced utilization of glucose. Haymond et al performed sequential glucose flux studies in 11 children (five control, six with epilepsy) and 10 adult volunteers using tagged glucose (16). All subjects were studied after a fast while either consuming a normal diet or the ketogenic diet. The authors found that glucose flux and ketonemia were inversely related, particularly when corrected for estimated brain mass. This was consistent with the replacement of glucose by ketone bodies for cerebral metabolism.
2. TCA cycle flux is increased by chronic ketosis. The enzyme alpha-ketoglutaric dehydrogenase is the rate-limiting enzyme in the TCA cycle function. The major inhibitor of this enzyme is the concentration of the product of the reaction, succinyl-CoA. This product is relatively reduced in the fat-fed group (down 26%), implying that the enzyme, alpha-ketoglutaric dehydrogenase, should be functioning at maximal capacity. Yet, concentrations of alpha-ketoglutarate, the substrate for this enzyme was found to be elevated 11% in the fat-fed group. The most plausible explanation is that the TCA cycle is driven to its maximal capacity, and overwhelms the ability of this rate-limiting enzyme to deal with the substrate. More recently, there has been renewed interest in anaplerosis or the repletion of TCA intermediates (17). The balance of TCA anions entering and leaving the cycle may serve as an important gateway in cell metabolism, and some have postulated that the anaplerotic impact of compounds might be another strategy for the management of seizures (18).
3. Cerebral energy reserves are increased by chronic ketosis.
Cerebral energy reserves were calculated from these measurements using the following equation:
Energy reserve = (PCr + ADP) + 2(ATP + glucose) + 2.9(glycogen) (19)
Applying the values determined for PCr, ADP, ATP, glucose, and glycogen enabled the calculation of the reserves. Energy reserves were significantly higher in the fat-fed rats (26.4 ± 0.6) compared to controls (23.6 ± 0.2) (P < .005).
Ketone bodies are thermodynamically more efficient fuels than glucose, because they avoid the less-efficient glycolytic pathway (Figure 70.2). Animals fed an equivalent amount of calories in the form of high-fat diets would therefore be expected to have more efficient energy production. Assuming that energy demands do not substantially change during fasting, the increased efficiency of ketone body utilization most likely contributes to higher energy reserves.
Pan et al used 31P spectroscopic imaging at 4.1T to demonstrate an elevated ratio of phosphocreatine/inorganic phosphorus in patients on the ketogenic diet and concluded that there was improvement of energy metabolism with use of the diet (20). Seven patients with intractable epilepsy (four with Lennox–Gastaut syndrome, one with absence seizures, one with primary generalized tonic–clonic seizures, and one with partial complex seizures) were studied before and after institution of the ketogenic diet. Coronal 1H anatomic imaging was performed to provide a correlate to the 31 P data. Ratios of phosphocreatine (PCr)/ATP were measured at baseline and compared with those obtained after the ketogenic diet. These showed a small but significant increase from 0.61 ± 0.08 to 0.69 ± 0.08 (P < .05). The ratio of PCr to inorganic phosphorus also changed from 2.45 ± 0.27 at baseline to 2.99 ± 0.44 during the diet (P < .05). The authors calculated an increase in the ∆G of ATP hydrolysis from 12.5 kcal/mol to 12.85 kcal/mol, or 2.5%.
In summary, the available biochemical data suggest that the KD favorably influences cerebral energetics by increasing cerebral energy reserves. This may be an important mechanism behind the increased resistance to seizures in ketotic brain tissue and the favorable cognitive effect sometimes seen with the ketogenic diet.
Direct Anticonvulsant Effects of Ketone Bodies
Ketone bodies have direct anticonvulsant effects, which perhaps relate to the chemical structural similarities of GABA, beta-hydroxybutyrate, and acetoacetate (Figure 70.3). Rats given intraperitoneal acetone showed reduction in seizures in the maximal electroshock, pentylenetetrazole, generalized kindled seizures, focal kindled seizures, and AY-9944 models (22). Acetoacetate and acetone also suppressed seizures in Frings audiogenic seizure-susceptible mice (22).
FIGURE 70.2 Summary of the relevant metabolic pathways affected by the ketogenic diet. The arrows ↑ and ↓ indicate significant differences of brain metabolites between ketotic animals and nonketotic animals. A, phophofructokinase; B, pyruvate dehydrogenase; C, α-ketoglutarate dehydrogenase; D, 3-oxoacid-CoA transferase; TCA, ticarboxylic acid; SSA, succinic semialdehyde. The biochemical observations imply that cerebral energetics is improved by chronic ketosis. These findings also suggest that flux rates through the ticarboxylic acid cycle and the gamma-aminobutyrate (GABA) shunt may be maximized and that GABA effects may be augmented.
Source: From Ref. (21). Nordli DR Jr, De Vivo DC. The ketogenic diet revisited: back to the future. Epilepsia. 1997;38:743–749. With permission.
FIGURE 70.3 Chemical structural similarities of GABA, acetoacetate, γ-hydroxybutyrate, and β-hydroxybutyrate.
Possible Neuroprotective Effects Mediated by Mitochondrial Uncoupling Protein
In a model thought to mimic human idiopathic epilepsy, EL mice were shown to have a delayed development of seizures, implying an antiepileptogenic effect of the diet (23). This, and the results of other studies, also suggest a possible neuroprotective effect of the diet, perhaps involving inhibition of caspase-3-mediated apoptosis (23,24). Others have examined the effect of the ketogenic diet upon mitochondrial uncoupling protein in the hippocampi of juvenile mice. Mitochondrial respiration rates were found to be substantially higher, and Western blots showed significant increases in uncoupling protein levels, particularly in the dentate gyrus of KD-fed mice. This, combined with the results of reactive oxygen species studies, suggested that the KD might be neuroprotective by diminishing reactive oxygen species production through activation of mitochondrial uncoupling proteins (24).
Other Mechanisms
A recent comprehensive review of the mechanisms of the ketogenic diet highlighted other possible actions (6). There is a possible neuroprotective effect of polyunsaturated fatty acids, which may help to reduce seizures. Medium chain fatty acids may have an inhibitory effect on hyperexcitability. There is evidence that ketone bodies induce neuroprotective signaling pathways through G protein-coupled receptors. Finally, inhibition of lactate dehydrogenase may lead to activation of K ATP channels.
Initiation of Therapy
Prior to initiation of the diet, a nutrition support team or registered dietician (RD) performs a comprehensive assessment. The nutritionist or dietician asks if there have been any gastrointestinal problems, food allergies, or feeding difficulties, such as problems with sucking, swallowing, or chewing. They also note the patient’s weight, height, usual weight, weight pattern since birth, and head circumference. Next, weight-for-age and height-for-age are plotted and ideal body weight-for-height is determined. Laboratory data are used as another tool for nutritional assessment of the patient, and we routinely obtain serum protein, lipid profile, electrolytes, free and total carnitine, hemoglobin, hematocrit, and red blood indices.
The nutritionist or dietician reviews the method of delivery of the diet, taking into consideration factors such as the patient’s age, stage of development, and expected tolerance of the regimen. In the usual case, the diet is offered in a normal, by-mouth fashion, with the expectation being that the child will eat and drink as is appropriate for his or her developmental stage. The consistency of the diet can be altered to adjust for any feeding difficulties. In very rare circumstances, the nutritional support team may recommend the use of a feeding tube.
If patients are to fast they should be hospitalized for the initiation of the ketogenic diet. Close observation is important because a child with an occult underlying inborn error of metabolism, particularly one that interfered with utilization of ketone bodies, could quickly decompensate (25). The hospitalization also provides the opportunity for family members to be instructed on maintenance of the diet.
While this is the traditional method of starting the diet for many, others argue that a fast is not necessary and that equivalent or superior results can be obtained without fasting (26). In one study, Bergqvist et al randomized children who were starting the ketogenic diet into two groups: one group began with a 24- to 48-hour fast, and the other had a gradual initiation into the diet without a fast (27). They found no difference in efficacy between the two groups, but those who had a gradual initiation into the diet without fasting had less weight reduction, fewer episodes of hypoglycemia, fewer treatments for acidosis, and a reduced need for IV fluid treatment for dehydration.
The LCT diet consists of three or four parts fat to one part nonfat (carbohydrate and protein) calculated on the basis of weight. It is computed to provide 75 to 100 kcal/kg-body weight and 1 to 2 g dietary protein per kg of body weight per day. Caloric requirements are adjusted to minimize weight gain and to maximize ketonemia. If a 3:1 (fat-to-nonfat) ratio is insufficient to produce the required ketosis, then a ratio of 4:1 is used.
The Conventional Ketogenic Diet or Long-Chain Triglyceride Diet
Prior to initiating the conventional ketogenic or LCT diet, a dietary prescription is made. Calculation of this prescription is straightforward. For example, if a 10 kg, 2-year-old child is to be started on a 3:1 diet, one begins by estimating the calorie requirements of the child:
10 kg × 100 kcal/day = 1,000 kcal/day
Alternatively, consulting a table of recommended daily allowances (RDAs) may derive this figure. In either case, it may require adjustment based upon the child’s individual metabolic needs. In general, prescriptions should start with 75% to 80% of RDA needs, but these figures may have to be modified depending upon the child’s level of activity.
The 3:1 ratio of the diet stipulates that 4 g of food must contain 3 g of fat and 1 g of nonfat. The nonfat consists of both carbohydrate (CHO) and protein. One gram of fat has the caloric equivalent of nine calories, whereas 1 g of protein or carbohydrate has the calorie equivalent of approximately four calories. Four g of food (arbitrarily referred to as one unit here) on a 3:1 diet is then equal to 31 calories:
1 g fat = 9 calories × 3 = 27 calories
1 g protein and CHO = 4 calories × 1 = 4 calories
Total calories = 27 + 4 = 31 calories per unit
To calculate the daily fat intake, one first divides the daily requirements of calories by this figure of 31 calories/unit, which generates the number of units required for the day:
1,000 calories/day/31 calories/unit = 32.25 units/day
Next, multiplying by 27 calories of fat/unit provides the daily fat requirement:
32.26 units/day × 27 calories of fat/unit = 871 calories of fat per day, which is equivalent to 96 g
The protein requirement depends upon the age of the child: children 1 to 3 years old require 1.2 g protein/kg/day, children 4 to 6 years of age require 1.1 g protein/kg/day, and children 7 and up require 1 g protein/kg/day. For this child the requirement would be 10 kg × 1.2 g/kg or 12 g/day (48 calories). Alternatively, one may consult the RDA table to determine the protein requirement.
So far, the combination of 871 calories of fat and 48 calories of protein leaves only 81 calories (1,000–919) not accounted for in the daily allowance. The carbohydrate intake is then calculated to supply the necessary remaining calories (81 calories), which here is approximately 20 g.
The diet prescription for this 10-kg patient on a 3:1 LCT diet is then:
Fat: 96 g per day or 32 g per meal
Protein: 12 g per day or 4 g per meal
Carbohydrate: 20 g per day or 7 g per meal
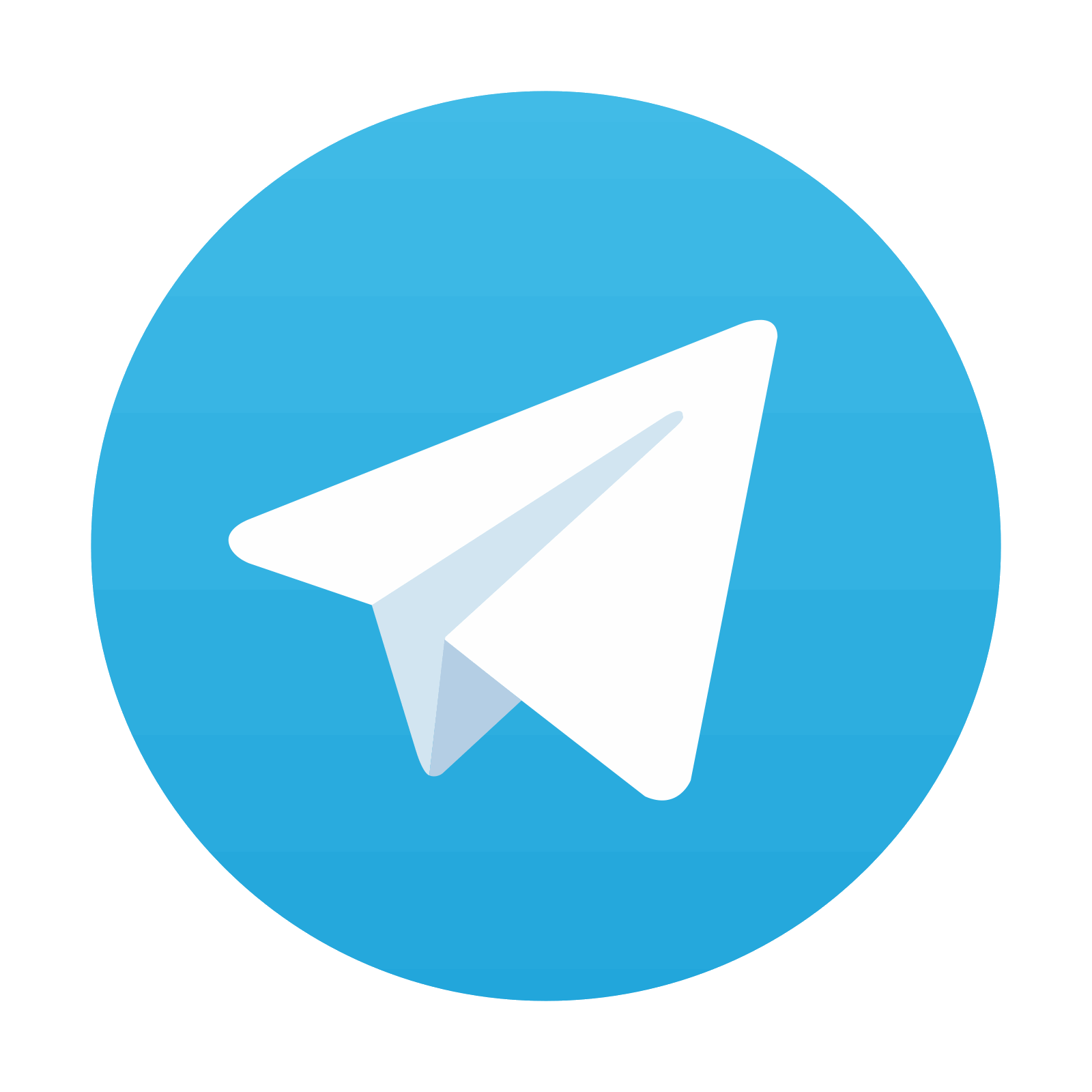
Stay updated, free articles. Join our Telegram channel
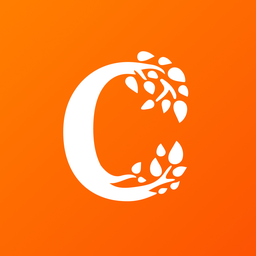
Full access? Get Clinical Tree
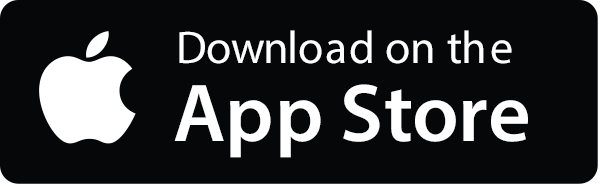
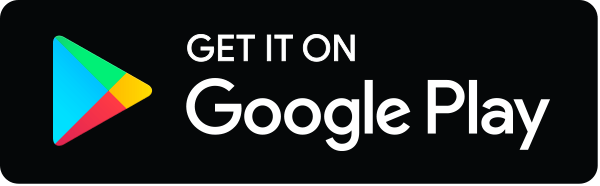