Key Abbreviations
Abdominal circumference AC
Absent end-diastolic velocity AEDV
Alpha-fetoprotein AFP
Amniotic fluid index AFI
Amniotic fluid volume AFV
Average for gestational age AGA
Biophysical profile BPP
Biparietal diameter BPD
Cerebroplacental Doppler ratio CPR
Computerized cardiotocography cCTG
Contraction stress test CST
Ductus venosus DV
Estimated date of confinement EDC
Femur length FL
Fetal activity count FAC
Fetal growth restriction FGR
Fetal heart rate FHR
Head circumference HC
Human chorionic gonadotropin hCG
Intrauterine growth restriction IUGR
Intraventricular hemorrhage IVH
Low birthweight LBW
Multiples of the median MoM
Necrotizing enterocolitis NEC
Neonatal intensive care unit NICU
Nonstress test NST
Nucleated red blood cell NRBC
Placental growth factor PlGF
Polymerase chain reaction PCR
Pregnancy-associated plasma protein A PAPP-A
Respiratory distress syndrome RDS
Reversed end-diastolic velocity REDV
Selective intrauterine growth restriction sIUGR
Small for gestational age SGA
Sonographically estimated fetal weight SEFW
Systolic/diastolic ratio S/D
Transcerebellar diameter TCD
The identification of pregnancies at risk for preventable perinatal handicap is a primary goal of the obstetric care provider. Pregnancies in which adverse intrauterine conditions result in failure of the fetus to reach its growth potential constitute such a high-risk group. Next to prematurity, intrauterine growth restriction (IUGR) is the second leading cause of perinatal mortality. Compared with appropriately grown counterparts, perinatal mortality rates in growth-restricted neonates are 6 to 10 times greater; perinatal mortality rates as high as 120 per 1000 for all cases of IUGR and 80 per 1000 after exclusion of anomalous infants have been reported. As many as 53% of preterm stillbirths and 26% of term stillbirths are growth restricted. In survivors, the incidence of intrapartum asphyxia may be as high as 50%. Prevention of some perinatal complications that lead to adverse outcomes in growth-restricted fetuses is possible with appropriate prenatal identification and management. This chapter reviews normal and disturbed fetal growth, the definition of abnormal fetal growth, the impact of fetal growth restriction, and the incorporation of this knowledge into screening, diagnosis, and management of these high-risk pregnancies. Intrauterine growth restriction (IUGR), fetal growth restriction (FGR), and small for gestational age (SGA) are terms frequently used interchangeably when describing the small fetus.
Regulation of Fetal Growth
Fetal growth is regulated at multiple levels and requires successful development of the placental interface between maternal and fetal compartments. In the early first trimester, anchoring villi that originate from the cytotrophoblast connect the decidua to the uterus and thereby establish placental adherence. This allows formation of vascular connections between the maternal circulation and the intervillous space so that increasing quantities of placental secretory products can reach the maternal circulation (see Chapter 1 ).
The villous trophoblast becomes the primary placental site of maternal-fetal exchange. By 16 weeks’ gestation, the maternal microvillous and fetal basal layer are only 4 microns apart, posing little resistance to passive diffusion. Elaboration of active transport mechanisms for three major nutrient classes—glucose, amino acids, and free fatty acids—and an increase in the villous surface area raise the capacity and efficiency of active transplacental transport. Vascular throughput across the placenta also increases in the maternal and fetal compartments. Extravillous cytotrophoblast invasion of the maternal spiral arteries results in progressive loss of the musculoelastic media, a process paralleled on the fetal side by continuous villous vascular branching. This results in significant reduction of blood flow resistance in the uterine and umbilical vessels, which converts both circulations into low-resistance, high-capacitance vascular beds. Owing to these developments, as much as 600 mL/min of maternal cardiac output reaches a placental exchange area of up to 12 m 2 at term. In the fetal compartment this is matched with a blood flow volume of 200 to 300 mL/kg/min throughout gestation. This magnitude of maternal blood flow is necessary to ensure maintenance of placental function that is energy intensive and consumes as much as 40% of the oxygen and 70% of the glucose supplied. Optimal fetal growth and development depends on a magnitude of maternal nutrient and oxygen delivery to the uterus that leaves sufficient surplus for fetal substrate utilization.
Of the actively transported primary nutrients, glucose is the predominant oxidative fuel, whereas amino acids are major contributors to protein synthesis and muscle bulk. Glucose and, to a lesser extent, amino acids drive the insulin-like growth factors axis and therefore stimulate longitudinal fetal growth.
Concurrent development and maturation of the fetal circulation as a conduit for nutrient and waste delivery allows preferential partitioning of nutrients in the fetus. Nutrient- and oxygen-rich blood from the primitive villous circulation enters the fetus via the umbilical vein. The ductus venosus (DV) is the first vascular partitioning shunt encountered. Through modulation in DV shunting, the proportion of umbilical venous blood distributed to the liver and heart changes with advancing gestation. Near term, 18% to 25% of umbilical venous flow shunts through the DV to reach the right atrium in this high-velocity stream; 55% reaches the dominant left hepatic lobe, and 20% reaches the right liver lobes ( Fig. 33-1 ). The differences in direction and velocity of blood entering the right atrium ensures that nutrient-rich blood is distributed to the left ventricle, myocardium, and brain while low-nutrient venous return is distributed to the placenta for reoxygenation and waste exchange. This process of blood distribution is referred to as preferential streaming . In addition to this overall distribution of left- and right-sided cardiac output, several organs can modify local blood flow to meet oxygen and nutrient demands by autoregulation.

When the milestones in maternal, placental, and fetal development are met, placental and fetal growth progress normally. The metabolic and vascular maternal adaptations promote a steady and enhanced nutrient delivery to the uterus, and placental transport mechanisms allow for efficient bidirectional exchange of nutrients and waste. Placental and fetal growth across the three trimesters are characterized by sequential cellular hyperplasia, hyperplasia plus hypertrophy, and lastly, by hypertrophy alone. Placental growth follows a sigmoid curve that plateaus in midgestation preceding exponential third-trimester growth of the fetus. During this exponential fetal growth phase of 1.5% per day, initial weight gain is due to longitudinal growth and muscle bulk and therefore correlates with glucose and amino acid transport. Eighty percent of fetal fat gain is accrued after 28 weeks’ gestation, providing essential body stores in preparation for extrauterine life. From 32 weeks onward, fat stores increase from 3.2% of fetal body weight to 16%, which accounts for the significant reduction in body water content.
Several possible mechanisms may challenge compensatory capacity of the maternal-placental-fetal unit to such an extent that failure to reach the growth potential may be the end result.
Definition and Patterns of Fetal Growth Restriction
Normal fetal growth involves hyperplasia and hypertrophy on a cellular level. Disturbance of fetal growth dynamics can lead to a reduced cell number, cell size, or both, ultimately resulting in abnormal weight, body mass, or body proportion at birth. The classification of abnormal growth has evolved significantly over the last century. From its modern origins in 1919 when Yippo was the first to label neonates with a birthweight below 2500 g as “premature” to the description of individualized growth potential, the recognition of the SGA neonate has advanced significantly. With the advent of ultrasound, the recognition and study of fetal growth abnormalities extended into the prenatal period. It is important to note that several definitions that describe abnormal growth are used interchangeably in the obstetric and pediatric literature, but they do not necessarily describe the same population.
The terminology for classifying fetal growth disorders has specifically expanded over the last four decades, which has led to confusion among the various terms used. From the 1960s, growth has been classified by the absolute birthweight value as low birthweight (LBW; <2500 g), very low birthweight (VLBW; <1500 g), extremely low birth weight (ELBW; <1000 g), or macrosomia (>4000 g). Subsequently, studies by Lubchenco, Usher, Battaglia, and others demonstrated that only the comparison of the actual birthweight to the expected weight in a population at the same gestational age identified small neonates at risk for adverse outcome. Adopting this concept of “light” and “heavy” for gestational age served as an introduction of population-based reference ranges for birthweight in the 1970s and allowed classification of growth by the birthweight percentile. This resulted in the currently accepted classification of birthweight as very small for gestational age (VSGA; <3rd percentile), small for gestational age (SGA; <10th percentile), average for gestational age (AGA; 10 th to 90th percentile), or large for gestational age (LGA; >90th percentile). Although birthweight percentiles are superior in identifying the small neonate, they fail to account for proportionality of growth and the growth potential of the individual. Therefore neonates with a normal birthweight percentile but abnormal body proportion as a result of differential growth delay may be missed. Similarly, birthweight percentiles do not distinguish between the small neonate normally grown given his or her genetic potential and the neonate that is growth restricted because of a disease process.
The detection of abnormal body mass or proportions is based on anthropometric measurements and ratios that are relatively independent of sex and race and, to a certain extent, gestational age and therefore also the traditional birthweight percentiles. The ponderal index ([birthweight in grams/crown heel length] 3 × 100) has a high accuracy for the identification of SGA and macrosomia. The ponderal index correlates more closely with perinatal morbidity and mortality than traditional birthweight percentiles, but it may miss the proportionally small and lean growth-restricted neonate. Several studies are currently underway to determine the most appropriate statistical methods to calculate the personalized growth potential across ethnicities. It is anticipated that these will improve the accurate prenatal identification of the SGA fetus.
The classification of abnormal growth during fetal life marks a significant advance because it opens the possibility of prenatal detection that leads to preventive or therapeutic management. The concept of percentiles has been adapted to the ultrasound biometry of the fetus. Accordingly, parameters of head, abdominal, and skeletal growth are related to population-based reference ranges. The prenatal diagnosis of FGR based on ultrasound biometry is described in detail below.
In addition to the detection of smaller individual measurements and lower weight, two principal patterns of disturbed fetal growth have been described: asymmetric and symmetric. In the asymmetric growth pattern, somatic growth (e.g., the abdominal circumference [AC] and lower body) shows a significant delay, whereas there is relative or absolute sparing of head growth. In the symmetric growth pattern, body and head growth are similarly affected. Asymmetric growth patterns result from two processes. First, liver volume is reduced because of depletion of glycogen stores as the result of limited nutrient supply, which leads to a decrease in AC. Second, elevations in placental blood flow resistance increase right cardiac afterload and promote diversion of the cardiac output toward the left ventricle because of the parallel arrangement of the fetal circulation and the presence of central shunts. Blood and nutrient supply to vital structures in the upper part of the body thus increase, presumably resulting in relative “head sparing.” Symmetrically restricted fetal growth typically results when interference with the growth process results in decreased cell numbers and cell size, typically resulting from a first-trimester insult. As a result, all parts of the body are equally affected, which produces uniformly small growth.
The pattern of fetal growth depends on the underlying cause of growth delay and on the timing and duration of the insult. Uteroplacental insufficiency is typically associated with asymmetric fetal growth delay owing to the aforementioned mechanisms. Aneuploidy, nonaneuploid syndromes, and viral infections either disrupt the regulation of growth processes or interfere with growth at the stage of cell hyperplasia. This typically results in a symmetric growth delay. Specific conditions such as skeletal dysplasia may produce distinct growth patterns by their differential impacts on axial and peripheral skeletal growth. Because growth is dynamic, the pattern of growth restriction may evolve in the course of gestation. Placental disease may initially present with relative head sparing but eventually progresses to symmetric growth delay as placental insufficiency worsens. Alternatively, the acute course of a fetal viral illness may temporarily result in arrested growth with subsequent resumption of a normal growth pattern.
Although the definition and classification of FGR has significantly evolved, research is still ongoing. Small fetal and neonatal sizes have to be considered as a physical sign rather than a specific disease that warrants further investigation of underlying causes. Given that the term small for gestational age simply identifies a fetus below a specific weight cutoff without identifying any underlying cause, the SGA designation will be used for this chapter when no underlying cause is evident. The terms intrauterine growth restriction and fetal growth restriction imply that some pathologic process has prohibited attainment of genetic growth potential, and these terms will be used accordingly in this chapter with a focus on underlying placental pathology. From the perspective of the managing obstetrician, prenatal identification of IUGR is most relevant because it allows appropriate prospective fetal management. Conversely, prenatal identification of the constitutionally small fetus will allow the obstetrician to reassure the patient. To formulate a uniform approach to prenatal detection and perinatal management, an understanding of the maternal and fetal impact of placental insufficiency is essential.
Etiologies of Intrauterine Growth Restriction
The precise mechanisms by which various conditions interfere with normal placentation and culminate in either pregnancy loss or IUGR are of great importance. Conditions that result in FGR broadly consist of maternal, uterine, placental, and fetal disorders. These conditions result in growth delay by affecting nutrient and oxygen delivery to the placenta (maternal causes), nutrient and oxygen transfer across the placenta (placental causes), and fetal nutrient uptake or regulation of growth processes (fetal causes). In clinical practice, considerable overlap may occur between the conditions that determine manifestation, progression, and outcome.
Maternal causes of FGR include vascular disease such as hypertensive disorders of pregnancy, diabetic vasculopathy, collagen vascular disease, and thrombophilia and chronic renal disease. Abnormalities of the fetus and/or placenta can also result in FGR. Chromosomal abnormalities, congenital malformations, and genetic syndromes have been associated with less than 10% of cases of IUGR. Similarly, although long recognized as a cause of growth restriction, intrauterine infection also accounts for less than 10% of all cases. However, genetic and infectious etiologies are of special importance because perinatal and long-term outcomes are ultimately determined by the underlying condition, with little potential impact through perinatal interventions.
Growth restriction has been observed in 53% of cases of trisomy 13 and in 64% of cases of trisomy 18 and may be manifest as early as the first trimester. Other conditions that may present with FGR include skeletal dysplasia and de Lange syndrome. The online database of inheritance in man lists over 100 genetic syndromes that may be associated with FGR. Of the infectious agents, herpes, cytomegalovirus (CMV), rubella, and Toxoplasma gondii are well-documented causes of symmetric IUGR.
The common causes of IUGR are listed in Box 33-1 . Recognition of these is important in planning the diagnostic workup for specific underlying conditions.
Maternal
- •
Hypertensive disease
- •
Pregestational diabetes
- •
Cyanotic cardiac disease
- •
Autoimmune disease
- •
Restrictive pulmonary disease
- •
High altitude (>10,000 feet)
- •
Tobacco/substance abuse
- •
Malabsorptive disease/malnutrition
- •
Multiple gestation
Fetal
- •
Teratogenic exposure
- •
Fetal infection
- •
Genetic disorders
- •
Structural abnormalities
Placental
- •
Primary placental disease
- •
Placental abruption and infarction
- •
Placenta previa
- •
Placental mosaicism
Although it is beyond the scope of this chapter to discuss the spectrum of clinical consequences, it needs to be recognized that the diagnosis and prognosis of FGR in twin pregnancies is critically determined by the chorionicity (see Chapter 32 ). Selective intrauterine growth restriction (sIUGR) is a specific term applied to FGR that occurs in monochorionic twins. The prognosis and risk profile can be determined based on the umbilical artery flow pattern.
Maternal and Fetal Manifestations of Intrauterine Growth Restriction
The impact and clinical manifestations of placental insufficiency depend on the gestational age at onset and the severity and type of the placental disease. Early interference with normal placentation affects all levels of placental and fetal development and culminates in the most severe clinical picture, which may result in miscarriage or early stillbirth. If sufficient supply to the placental mass can be established, further placental differentiation may be possible, but suboptimal maternal adaptation to pregnancy and deficient nutrient delivery pose limitations. If adaptive mechanisms permit ongoing fetal survival, early-onset growth restriction with its many fetal manifestations develops. If placental disease is mild or successful compensation has occurred, the consequences of nutrient shortage may remain largely subclinical, only to be unmasked through their restrictive effect on exponential fetal growth in the second to third trimester. In these cases, late-onset growth delay, a decrease in adipose tissue, or abnormal body proportions at birth may be the consequence.
Maternal Impacts
Placental dysfunction affects several aspects of maternal adaptation to pregnancy. Associations between poor placentation with suboptimal maternal volume expansion, increased vascular reactivity, and a “flat” curve on the glucose tolerance test have been described. Abnormalities of placental vascular development are of special interest because they can be detected by Doppler ultrasound of the uterine arteries and frequently predate clinical disease ( Fig. 33-2 ). When trophoblast invasion remains confined to the decidual portion of the myometrium, maternal spiral and radial arteries fail to undergo the physiologic transformation into low-resistance vessels, which is generally expected by 22 to 24 weeks. Maternal placental floor infarcts, fetal villous obliteration, and fibrosis each increase placental blood flow resistance, producing a maternal-fetal placental perfusion mismatch that decreases the effective exchange area. With progressive vascular occlusion, fetoplacental flow resistance is increased throughout the vascular bed, and eventually, metabolically active placental mass is reduced. The diagnostic and screening utility of ultrasound findings suggestive of such pathology are discussed below.

Fetal Impacts
When placental dysfunction compromises nutrient delivery and triggers fetal mobilization of hepatic glycogen stores, physical manifestation of growth delay becomes clinically apparent. In addition to the cardinal sign of FGR, metabolic, endocrine, hematologic, cardiovascular, and behavioral manifestations of placental insufficiency have been described that relate to the severity and duration of placental dysfunction. Of these, cardiovascular and central nervous system (CNS) responses are best studied because their noninvasive assessment is readily achieved by multivessel Doppler, gray-scale ultrasonography, and fetal heart rate (FHR) analysis and can therefore be utilized for fetal surveillance. Appreciating the variety of fetal manifestations helps to understand the potential limitations of antenatal surveillance and provides a basis on which to appreciate the possible short- and long-term impacts of placental insufficiency.
Metabolic manifestations occur early in growth-restricted fetuses. This is because with mild to moderate decline in uterine nutrient delivery, fetal supply is compromised first, whereas placental nutrition is preferentially maintained. With progression of placental insufficiency, nutrient deficits become universal and result in decreased fetal and placental size. Accordingly, with mild restrictions in oxygen and glucose supply, fetal demands are still met by increased fractional extraction. When uterine oxygen delivery falls below a critical value (0.6 mmol/min/kg fetal body weight in sheep), fetal oxygenation begins to fall and is eventually accompanied by fetal hypoglycemia. The initially mild hypoglycemia results in a blunted fetal pancreatic insulin response that allows gluconeogenesis from hepatic glycogen stores. The minimal hepatic glycogen stores in the fetus are quickly depleted as glucose and lactate are preferentially diverted to the placenta. An increasing nutrient deficit leads to worsening fetal hypoglycemia, which decreases the maintenance of fetal oxidative metabolism and placental nutrition. With a more significant limitation of oxidative metabolism associated with downregulation of placental transport mechanisms and intensifying hypoglycemia, the use of other fetal energy sources becomes necessary, and more widespread metabolic consequences ensue. Limitation of amino acid transfer and breakdown of endogenous muscle protein to obtain gluconeogenic amino acids depletes branched-chain and other essential amino acids. Simultaneously, lactate accumulates because of the limited capacity for oxidative metabolism. Placental transfer of fatty acids loses its selectivity, particularly for essential fatty acids. Reduced utilization leads to increased fetal free fatty acid and triglyceride levels with a subsequent failure to accumulate adipose stores. In this setting of advancing malnutrition, cerebral and cardiac metabolism of lactate and ketones is upregulated to remove these accumulating products of anaerobic metabolism. Acid-base balance can be maintained as long as acid production is met by sufficient buffering capacity of fetal hemoglobin and an equal disposition by the various organs. Thus metabolic compromise progresses from simple hypoglycemia, hypoxemia, and decreased levels of essential amino acids to overt hypoaminoacidemia, hypercapnia, hypertriglyceridemia, and hyperlacticemia. The lactate production is exponentially correlated to the degree of acidemia that generally results from this metabolic state. A summary of metabolic responses is provided in Table 33-1 .
Substrate | Change |
Glucose | Decreased proportional to the degree of fetal hypoxemia |
Amino acids | Significant decrease in branched-chain amino acids (valine, leucine, isoleucine) as well as lysine and serine; in contrast, hydroxyproline is elevated. The decrease in essential amino acids is proportional to the degree of hypoxemia. Elevated amniotic fluid glycine to valine ratio Elevations in amniotic fluid ammonia with a significant positive correlation with the fetal ponderal index |
Fatty acids and triglycerides | Decrease in long-chain polyunsaturated fatty acids (docosahexaenoic and arachidonic acids), decrease in overall fatty acid transfer only with significant loss of placental substance Hypertriglyceridemia due to decreased utilization Lower cholesterol esters |
Oxygen and carbon dioxide | Degree of hypoxemia proportional to villous damage; correlates significantly with hypercapnia, acidemia and hypoglycemia, and hyperlacticemia |
Fetal endocrine manifestations of placental insufficiency are relevant because they are responsible for the downregulation of growth and developmental processes. Decreases in fetal glucose and amino acids indirectly downregulate insulin and insulin-like growth factors (IGFs) I and II as the principal endocrine regulators of longitudinal growth. Leptin-coordinated deposition of fat stores is similarly affected. In addition, pancreatic cellular dysfunction becomes evident through a decreased insulin/glucose ratio and impaired fetal glucose tolerance. Significant elevations of corticotropin-releasing hormone (CRH), adreonocorticotropic hormone (ACTH), and cortisol and a decline in active vitamin D and osteocalcin all correlate with the severity of placental dysfunction. These hormonal imbalances are believed to have additional negative impacts on linear and growth, bone mineralization, and the potential for postpartum catch-up growth.
In growth-restricted fetuses, declining function at all levels of the thyroid axis correlates to the degree of hypoxemia. Thyroid gland dysfunction may develop as indicated by low levels of thyroxine and triiodothyronine despite elevated thyroid-stimulating hormone (TSH) levels. In other instances, central production of TSH may be responsible for fetal hypothyroidism. Finally, downregulation of thyroid hormone receptors may limit the biologic activity of circulating thyroid hormones in specific target tissues such as the developing brain.
Elevations in serum glucagon, adrenaline, and noradrenaline and stimulation of the fetal glucocorticoid axis have immediate effects that promote the mobilization of hepatic glycogen stores and peripheral gluconeogenesis. However, persistent alterations of these hormones may have a causative relationship with the development of diabetes and vascular complications in adult life.
Fetal hematologic responses to placental insufficiency are important because they initially provide a compensatory mechanism for hypoxemia and acidemia but eventually become contributory to the escalation of placental vascular dysfunction. Fetal hypoxemia is a trigger for erythropoietin release and stimulation of red blood cell (RBC) production through both medullary and extramedullary sites, which results in polycythemia. Oxygen-carrying capacity and the buffering capacity are thus increased through the elevation in hemoglobin count. If extramedullary hematopoiesis is increasingly induced by prolonged tissue hypoxemia and/or acidosis, the nucleated red blood cell (NRBC) count rises owing to the escape of these cells from these sites. Thus elevated NRBC counts correlate with metabolic and cardiovascular status and are independent markers for poor perinatal outcome. In advanced placental insufficiency, more complex hematologic abnormalities supervene that may result from dysfunctional erythropoiesis, placental consumption of platelets, and vitamin and iron deficiency. Subsequently, fetal anemia and thrombocytopenia are observed, particularly in fetuses with marked elevation of placental blood flow resistance and evidence of intraplacental thrombosis, which suggests a causative relationship. Increase in whole blood viscosity, decrease in RBC membrane fluidity, and platelet aggregation may be important precursors in the acceleration of placental vascular occlusion and dysfunction.
Growth-restricted fetuses also show evidence of immune dysfunction at the cellular and humoral level. Decreases in immunoglobulin, absolute B-cell counts, total white blood cell (WBC) counts, and neutrophil, monocyte, and lymphocyte subpopulations—as well as selective suppression of T-helper and cytotoxic T cells—are all related to the degree of acidemia. These immune deficiencies explain the higher susceptibility of growth-restricted neonates to infection after delivery.
Fetal cardiovascular responses to placental insufficiency can be subdivided into early and late based on the degree of deterioration of cardiovascular status and the associated derangement of fetal acid-base balance (see below). Early responses are typically adaptive in nature and result in preferential nutrient streaming to essential organs. The combination of elevated placental blood flow resistance and impaired transplacental gas transfer has several effects on the fetal circulation. Normally, nutrient-rich oxygenated blood enters the fetus via the umbilical vein and reaches the liver as the first major organ. One of the earliest cardiovascular signs of placental insufficiency is a decrease in the magnitude of umbilical venous flow volume. In response to these changes in umbilical venous nutrition content and blood flow volume, the proportion of umbilical venous blood diverted through the ductus venosus toward the fetal heart increases. This change in venous shunting across the DV increases the proportion of nutrient-rich umbilical venous blood that bypasses the liver and reaches the left side of the heart through the foramen ovale. Because of the presence of central shunts at the level of the foramen ovale and ductus arteriosus, differential changes in downstream blood flow resistance can affect the proportion of cardiac output delivered through each ventricle ( Fig. 33-3 ). Elevation of blood flow resistance in the pulmonary vascular bed and subdiaphragmatic circulation (lower body and placenta) increase right ventricular afterload. A drop in cerebral blood flow resistance decreases left ventricular afterload. As a consequence, shunting of nutrient-rich blood from the DV through the foramen ovale to the left side of the heart increases, and left ventricular output rises in relation to the right cardiac output. At the aortic isthmus, blood coming from the right ventricle through the ductus arteriosus is diverted toward the aortic arch, thereby supplementing this central shift in cardiac output from right to left. This relative shift in cardiac output toward the left ventricle that results in increased blood flow to the myocardium and brachiocephalic circulation has been termed redistribution, which indicates a compensatory mechanism in response to placental insufficiency.

Late circulatory responses are associated with deterioration of cardiovascular status and are predominantly observed in early-onset growth delay, which requires delivery prior to 34 weeks. Redistribution is only effective as long as adequate forward cardiac function is maintained. Marked elevations in placental blood flow resistance and progressive placental insufficiency can lead to an impairment of cardiac function. When this occurs, several aspects of cardiovascular homeostasis can be affected. Ineffective preload handling and elevation in central venous pressure may result from ineffective redistribution, a measurable decline in cardiac output, and a decline in cardiac forward function. The hallmark of this advancing deterioration in cardiovascular state is loss of diastolic forward flow in the umbilical circulation and a marked decline of forward flow in the venous system. Finally, myocardial dysfunction and cardiac dilatation may result in holosystolic tricuspid insufficiency and spontaneous FHR decelerations, followed by fetal demise.
Fetal organs also have the ability to regulate their individual blood flow through autoregulation. Such autoregulatory mechanisms have been identified in the myocardium, adrenal glands, spleen, liver, celiac axis, mesenteric vessels, and kidneys. These autoregulatory mechanisms are evoked at different levels of compromise and their effect is typically complementary to central blood flow redistribution by enhancing perfusion of vital organs as long as cardiovascular homeostasis is maintained. A summary of Doppler findings and their physiologic significance in the context of placental insufficiency is provided in Table 33-2 .
Fetal behavioral responses to placental insufficiency and characteristics of the FHR reflect developmental status and undergo significant changes with advancing gestation. Progressive sophistication of fetal behavior and increasing variation of the FHR reflect differentiation of central regulatory centers and expansion of central processing capability. Normally, behavioral milestones progress from the initiation of gross body movements and fetal breathing in the first trimester to coupling of fetal behavior (e.g., heart rate reactivity) and integration of rest-activity cycles into stable behavioral states (states 1 through 4 F) by 28 to 32 weeks’ gestation (see Chapter 11 ). A steady decrease in baseline heart rate that reflects increasing vagal tone accompanies these developments. In addition, short- and long-term variability and variation, as well as the amplitude of accelerations, increase with advancing gestation, which reflects increased central processing. With the completion of these milestones, heart rate reactivity by traditional criteria is present in 80% of fetuses by 32 weeks’ gestation. Differences in maturational state and behavioral state, disruption of neural pathways, and declining oxygen tension may all modulate or even abolish fetal behavior or heart rate characteristics.
Because variations of fetal behavior and the FHR may be due to several factors, observation of several variables over a sufficient length of time is necessary to separate physiologic from pathologic variation. Biophysical profile (BPP) scoring quantifies fetal behavior by assessing tone, movement, breathing activity, and FHR reactivity in an observation period of at least 30 minutes (see Chapter 11 ). Amniotic fluid volume (AFV) assessment has traditionally been a part of the BPP. From the second trimester onward, AFV is primarily related to fetal urine production and therefore renal perfusion (see Chapter 35 ). Thus AFV assessment provides an indirect assessment of renal/vascular status and constitutes the main longitudinal monitoring component of the BPP (see below). Visual FHR analysis is associated with interobserver and intraobserver variability. These are circumvented by computerized cardiotocography (cCTG) analysis of the fetal heart rate. The cCTG assesses short-term, long-term, and mean minute variation and periods of high variation in addition to traditional FHR parameters that also allow longitudinal observations.
In growth-restricted fetuses with chronic hypoxemia and mild placental dysfunction, the primary CNS response is a delay in all aspects of CNS maturation. With the help of computerized research tools, a delay in behavioral development has been documented under such circumstances. The combination of delayed central integration of FHR control, decreased fetal activity, and chronic hypoxemia results in a higher baseline heart rate with lower short- and long-term variation (on computerized analysis) and delayed development of heart rate reactivity. These maturational differences in FHR parameters are particularly evident between 28 and 32 weeks.
Despite the maturational delay of some aspects of CNS function, several centrally regulated responses to acid-base status are still preserved. Therefore the growth-restricted fetus still maintains behavioral responses to a decline in acid-base status independently of the cardiovascular status. In contrast, the declining AFV that commonly accompanies the sequential loss of biophysical variables appears to be related to renal blood flow and the degree of vascular redistribution .
With worsening fetal hypoxemia, a decline in global fetal activity initiates the cascade of late behavioral responses characteristic of placental insufficiency. With further deepening hypoxemia, fetal breathing movement ceases.
Gross body movements and tone decrease further until they are no longer observed in the traditional examination period. Traditional FHR variables are frequently abnormal by this time. Reduction of global fetal activity and loss of fetal coupling (absence of heart rate reactivity and fetal breathing movements) are typically observed at a mean pH between 7.10 and 7.20. Loss of tone and movement is characteristic as the pH drops further. Late decelerations of the FHR may develop because of a relative drop in oxygen tension that exceeds 8 torr (see Chapter 15 ). Spontaneous decelerations as a result of direct depression of cardiac contractility or “cardiac” late decelerations typically herald fetal demise.
Diagnostic Tools in Fetal Growth Restriction
Fetal growth restriction is a syndrome marked by failure of the fetus to reach its growth potential with consequences related to the underlying disorder as well as the severity of fetal disease. Because IUGR may be the consequence of many underlying etiologies, the differential diagnosis always includes maternal disease, placental insufficiency, aneuploidy, nonaneuploid syndromes, and viral infection. For appropriate patient counseling and choice of management options, comprehensive prenatal evaluation must go beyond the assessment of fetal size, utilizing a diagnostic approach aimed at identifying the underlying causes. After confirming small fetal size, stratification into three patient groups is of particular importance. The first group consists of constitutionally small but otherwise normal fetuses. These will not usually require any intervention and therefore do not need antenatal surveillance. The second group consists of fetuses with aneuploidy, nonaneuploid syndromes, or viral infection. In these conditions, prognosis is largely determined by the underlying disease, with little potential for impact by perinatal interventions. Sensitive and knowledgeable counseling of the parents about the likely prognosis in these conditions is especially important in such cases. The third group consists of fetuses with placental disease in which progressive deterioration of the fetal condition worsens the prognosis. This subset of patients is most likely to benefit from fetal surveillance and subsequent intervention. Although gray-scale ultrasonography provides important clues to the presence of IUGR, the liability of preterm delivery and iatrogenic complications is great if the diagnosis is based solely on biometry. Although maternal disease is readily apparent through a history and physical examination, the accurate evaluation of a possible fetal disorder and stratification of risk requires the integration of several diagnostic modalities that evaluate fetal, placental, and amniotic fluid characteristics.
Fetal Biometry
Ultrasound criteria have emerged as the diagnostic standard used in the identification of FGR. For this purpose, sonographic measurements of fetal bony and soft structures are related to reference ranges for gestation. The primary measurements utilized to evaluate fetal growth include those of the fetal head, abdominal circumference, and long bones. The most important calculated ultrasound variable of fetal growth is the sonographically estimated fetal weight (SEFW), and numerous investigators have identified distinct fetal ultrasound parameters that are useful in the calculation of the SEFW. All of the techniques incorporate an index of abdominal size as a variable contributing to the estimation of fetal weight. Population-specific formulae have been derived to generate reference limits that generally have 95% confidence limits that deviate approximately 15% around the actual value.
Accurate estimation of fetal growth from these fetal measurements requires knowledge of the gestational age as a reference point to calculate percentile ranks. An estimated date of confinement (EDC) should be based on the last menstrual period when the sonographic estimate of gestational age is within the predictive error (7 days in the first, 14 days in the second, and 21 days in the third trimester). Once the EDC is set by this method or by a first-trimester ultrasound, it should not be changed because such practice interferes with the ability to diagnose IUGR.
Measurement of the biparietal diameter (BPD) alone is a poor tool for the detection of IUGR. The physiologic variation in size inherent with advancing gestation is high. The majority of growth-restricted fetuses who present with asymmetric growth restriction and delayed flattening of the cranial growth curve would be detected relatively late. Factors that interfere with a technically adequate measurement of the BPD include alterations of the cranial shape by external forces (oligohydramnios, breech presentation) and direct anteroposterior position of the fetal head.
The head circumference (HC) is not subject to the same extrinsic variability as the BPD. The measurement technique is important because calculated HC measurements are systematically smaller than those directly measured, thus the nomogram selected should be based on measurements obtained using the same methodology. As a screening tool for IUGR, the HC poses a similar problem as the BPD in that two thirds of IUGR fetuses with asymmetric growth pattern would be detected late.
The transcerebellar diameter (TCD) is one of the few soft tissue measurements that correlate well with gestational age, being relatively spared from the effects of mild to moderate uteroplacental dysfunction. Whether its measurement offers any advantage over bony measurements in the assessment of compromised fetal growth is controversial.
The AC is the single best measurement for the detection of IUGR. The most accurate AC is the smallest directly measured circumference obtained in a perpendicular plane of the upper abdomen at the level of the hepatic vein between fetal respirations. The AC percentile has both the highest sensitivity and negative predictive value for the sonographic diagnosis of IUGR whether defined postnatally by birthweight percentile or ponderal index. Using the 10th percentiles as cutoffs, the AC has a higher sensitivity (98% vs. 85%) but lower positive predictive value (PPV) than the SEFW (36% vs. 51%). Its sensitivity is further enhanced by serial measurements at least 14 days apart. Because of its high sensitivity, some type of abdominal measurement should be part of every sonographic growth evaluation. However, because the AC reflects fetal nutrition, it should be excluded from the calculation of the composite gestational age after the early second trimester.
Calculation of the ratio between the head and abdominal circumference (HC/AC ratio) has been proposed as a tool to increase detection of the fetus with asymmetric growth restriction ( Figs. 33-4 and 33-5 ). In the normally growing fetus, the HC/AC ratio exceeds 1.0 before 32 weeks’ gestation, is approximately 1.0 at 32 to 34 weeks’ gestation, and falls below 1.0 after 34 weeks’ gestation. In fetuses with asymmetric growth restriction, the HC remains larger than that of the body and results in an elevated HC/AC ratio, whereas the ratio remains normal in symmetric IUGR, in which both direct measurements are equally affected (see Figs. 33-4 and 33-5 ). Using the HC/AC ratio, 70% to 85% of growth-restricted fetuses are detected, with a reduction in false-negative diagnoses. Thus even when determined in the latter part of pregnancy, a single set of measurements can be very helpful in evaluating the status of fetal growth. However, both the sensitivity and the PPV of the HC/AC ratio for growth restriction does not equal either the AC percentile or the SEFW.


When measurement of the HC is difficult because of fetal position, comparisons can be made of the femur length (FL)—which is relatively spared in asymmetric IUGR—to the AC. The FL/AC ratio is 22 at all gestational ages from 21 weeks to term; therefore this ratio can be applied without knowledge of the gestational age. An FL/AC ratio greater than 23.5 suggests IUGR.
Several formulae have been devised to calculate the SEFW. Using a multifactorial equation and a measurement of abdominal size, a weight can be predicted and related to gestational age. Formulae that incorporate the FL may increase the accuracy of in utero weight estimation for the fetus with IUGR. Because the SEFW cannot be measured directly but is calculated from a combination of directly measured parameters, the error in the estimate is increased. The accuracy of most formulae (±2 standard deviations [SDs]) is ± 10%, and none has proven superior to the first devised by Warsof and reported by Sheppard. As noted earlier, the SEFW has a lower sensitivity but higher PPV than the AC and does not add to the AC percentile for the diagnosis of IUGR. However, an SEFW below the 10th percentile provides a graphic image that makes it easy for both patient and referring physician to conceptualize. Therefore use of the estimated fetal weight (EFW) has become the most common method for characterizing fetal size and thereby growth abnormalities.
Reference Ranges That Define Fetal Growth
An absolute threshold to define IUGR can be applied to any of the fetal biometric parameters. These criteria have been statistically defined, rather than outcome based, and use either a threshold percentile ranking (nonnormative data) or a number of standard deviations below the mean (normative data) as cutoffs. Small for gestational age is defined as a birthweight below the population 10th percentile corrected for gestational age. However, this cutoff has also been the most widely used criterion for defining growth restriction at birth. This definition has also been adopted for the prenatal period by using an SEFW below the 10th percentile as an indicator of IUGR. Because such an approach is purely based on a weight threshold, it can only serve as a screen for the identification of the small fetus at risk for adverse outcomes. Approximately 70% of infants with a birthweight below the 10th percentile are normally grown (i.e., constitutionally small) and are not at risk for adverse outcomes because they present one end of the normal spectrum for neonatal size. The remaining 30% consist of infants who are truly growth restricted and are at risk for increased perinatal morbidity and mortality. When the cutoff for an abnormal birthweight is adjusted to the 3rd percentile, the proportion of true growth-restricted infants identified increases, whereas some with milder forms of IUGR will be missed. The principal advantage of a lower percentile cutoff is the identification of fetuses at greatest need for antenatal surveillance. This is further emphasized by the increased mortality for birthweights through the 15th percentile with an odds ratio of 1.9 for mortality in newborns with birthweights between the 10th and 15th percentiles. Because the percentile cutoffs to define abnormal growth continue to be a matter of debate, risk assessment based on weight alone is further hampered by discrepancies between the SEFW and the actual birthweight. Live birthweight criteria do not appropriately describe SEFWs, and a significant association of preterm birth with FGR is apparent. The weights of preterm infants are not normally distributed as they are at term, which produces a significant discrepancy between birthweight-defined growth curves and SEFW-defined growth curves in preterm gestation. SEFW growth curves are generated from patient samples that represent the entire obstetric population at any gestational age. In contrast, preterm live birth normative data tables reflect only those individuals who have delivered under abnormal circumstances. Thus the SEFW growth curves consistently demonstrate higher fetal weights over the range of preterm gestation than do birthweight-generated growth curves. Therefore use of an SEFW cutoff below the 10th percentile is more appropriate to define abnormal fetal growth because the ability to identify fetuses at greater perinatal risk is increased. For the AC, a cutoff of the 2.5 percentile is appropriate because reference ranges are based on a cross section of small, appropriately grown preterm and term newborns. However, because reference limits are based on healthy women delivering appropriately nourished neonates at term, less than the 10th percentile cutoff is consistent with IUGR.
Because of the limitation of population-based reference ranges to assess fetal growth , individualized growth models have been proposed by several investigators. The obvious advantage is the lack of dependency on population-based normative data and the ability to detect a true, singularly defined growth restriction even with EFWs above the 10th percentile for the population. Some of these models require three sequential sonograms. This includes baseline biometry in the second trimester, a second sonogram to establish growth potential for an individual morphometric parameter, and a third scan to identify a growth abnormality. Because this approach is cumbersome in clinical practice, other models have been developed that account for variables that contribute the majority of the variance to newborn size. These include early pregnancy weight, maternal height, ethnic group, parity, and sex. Using these variables and fetal growth patterns, the estimated size of a fetus of a given mother can be projected at term and estimated at any specific point in gestation. Deviations from this projected growth pattern can then be recognized. Overall, the diagnostic advantages of these individualized growth models has been questioned when compared with the sequential comparison of the percentile ranking of individual or composite growth parameters to population-based growth curves.
Fetal growth, as opposed to fetal size, is a dynamic process that requires more than a single evaluation for its estimation. The appropriate observation interval for the evaluation of fetal growth has been based on the assumptions that growth is continuous, rather than sporadic, and that the identification of growth is limited by the technical capability of the ultrasound equipment used to measure the fetus. The recommended interval between ultrasound evaluations of fetal growth is 3 weeks because shorter intervals increase the likelihood of a false-positive diagnosis.
In summary, the ability to correctly identify growth-restricted fetuses at risk for adverse outcome by weight estimates alone is limited. Individualized or sequential growth assessment performs better than a single measurement of fetal size. Improved stratification of risk requires the integration of additional diagnostic tests.
Fetal Anatomic Survey
The sonographic survey of fetal anatomy may provide a clue to the underlying etiology of IUGR. The anatomic survey should focus on markers of aneuploidy, nonaneuploid syndromes, and fetal infection as well as structural malformations. The relationship between aneuploidy and fetal anomalies such as omphalocele, diaphragmatic hernia, congenital heart defects, and sonographic markers such as echogenic bowel, nuchal thickening, and abnormal hand positioning are discussed in Chapter 9 . Abnormalities of skull contour, thoracic shape, or disproportional shortening of the long bones may be suggestive of a skeletal dysplasia. Markers for viral infection may be nonspecific but include echogenicity and calcification in organs such as the brain and liver. Identification of any of these abnormalities on ultrasound may help to establish a differential diagnosis and can impact the prognosis.
Amniotic Fluid Assessment
From the second trimester onward, regulation of AFV is primarily dependent on fetal urine output, production of pulmonary fluid, and fetal swallowing (see Chapter 35 ). Placental dysfunction and fetal hypoxemia both may result in decreased perfusion of the fetal kidneys with subsequent oliguria and decreasing AFV. Although the accuracy of ultrasound in the assessment of actual AFV is poor, two techniques are used that can provide important diagnostic and prognostic information. A vertical pocket of amniotic fluid measuring 1 cm or more was historically considered indicative of an adequate fluid volume. Criteria for AFV assessment were subsequently broadened. A 2-cm vertical pocket was considered normal, 1 to 2 cm was marginal, and less than 1 cm was decreased. Alternatively, AFV may be assessed by the sum of vertical pockets from the four quadrants of the uterine cavity. This four-quadrant amniotic fluid index (AFI) nomogram (see Fig. 33-3 ) is compared with gestational reference ranges. Despite the availability of these numerical methods for estimating the AFV, an overall clinical impression of reduced amniotic fluid may be most important. Ultrasound criteria for a subjectively reduced AFV include a maximum vertical pocket less than 3 cm, the fetus in a flexion attitude with limited room for movement, a small or empty bladder and stomach, and molding of the uterus around the fetal body. In addition, movement of the transducer frequently generates uterine contractions, which may be associated with variable decelerations.
Overall, estimation of AFV in itself is a poor screening method for IUGR or fetal acidemia. However, in clinical practice, it is an important diagnostic and prognostic tool. Oligohydramnios may be the first sign of FGR detected on ultrasonography preceding an assessment for lagging fetal growth. If gestational age is known, ultrasound assessment of fetal growth based on the HC, AC, FL, and SEFW can be performed. If gestational age is unknown, measurements of the FL/AC ratio and a single amniotic fluid pocket have to be used because they are independent of gestational age. Up to 96% of fetuses with fluid pockets less than 1 cm may be growth restricted. When growth delay is already suspected, assessments of AFV can help with a differential diagnosis. In the setting of small fetal size, abundant AFV suggests aneuploidy or fetal infection, whereas normal or decreased amniotic fluid is compatible with placental insufficiency. The volume of amniotic fluid also has prognostic significance for the course of labor. Groome and colleagues demonstrated that oligohydramnios associated with fetal oliguria is associated with a higher rate of intrapartum complications that may be attributed to reduced placental reserve.
Doppler Velocimetry
Similar to the assessment of AFV, the role of Doppler velocimetry in the management of FGR is unique because it serves as a diagnostic, as well as a monitoring, tool. Doppler flow-velocity waveforms may be obtained from arterial and venous vascular beds in the fetus. Arterial Doppler waveforms provide information on downstream vascular resistance, which may be altered because of structural changes in the vasculature or regulatory changes in vascular tone. The systolic/diastolic (S/D) ratio, the resistance index (RI), and the pulsatility index (PI) are the three Doppler indices most widely used to analyze arterial blood flow resistance (see Table 33-2 ). An increase in blood flow resistance manifests with a relative decrease in end-diastolic velocity that results in an increase in all three Doppler indices. Of these, the pulsatility index has the smallest measurement error and narrower reference limits. With extreme increases in blood flow resistance, end-diastolic forward flow velocity may be absent or reversed, referred to as absent end-diastolic velocity (AEDV) or reversed end-diastolic velocity (REDV; Fig. 33-6 ).

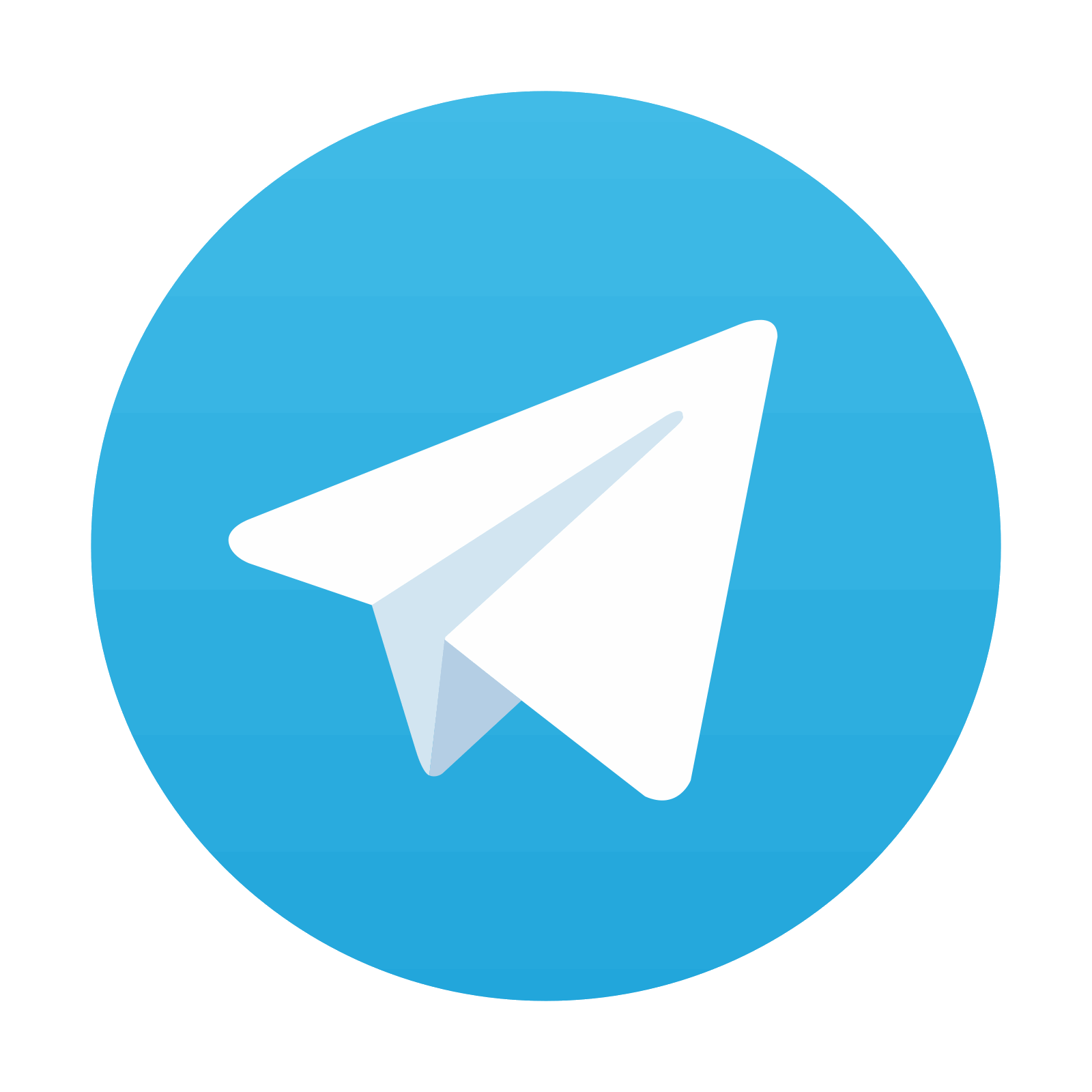
Stay updated, free articles. Join our Telegram channel
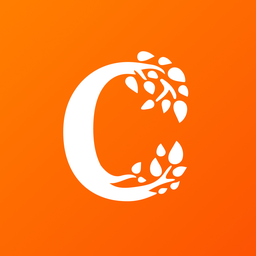
Full access? Get Clinical Tree
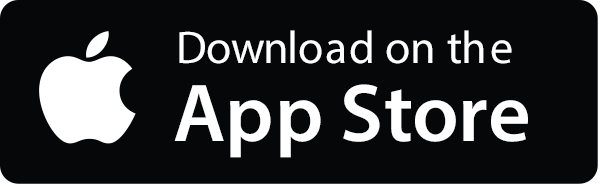
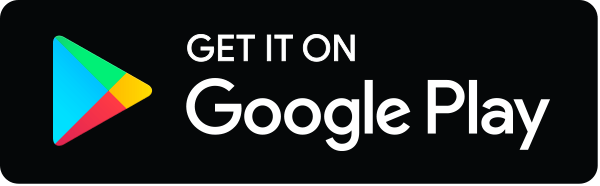