Insulin and Diabetes Mellitus
Tania S. Burgert
Eda Cengiz
William Tamborlane
Prior to the advent of insulin therapy, the life expectancy of children with type-1 diabetes mellitus (T1DM) was approximately 1 year from diagnosis. Referred to as the “Pissing Evile” in 17th-century England, this disease was known for its agonizing, unremitting progression to emaciation, coma, and death (1). The revolutionary discovery of insulin changed the diagnosis of diabetes from a slowly deteriorating death sentence to a full life of near limitless possibilities. Not surprisingly, the Nobel Prize in medicine was awarded to the discoverers of insulin just 1 year after its successful extraction from beef pancreas in 1922. This insulin preparation, containing numerous impurities and varying potency, was put in use immediately for the treatment of diabetes. Since then insulin has been on the forefront of science becoming one of the best-characterized vertebrate hormones, with a wealth of data on its crystalline structure and mechanism of action at the molecular, cellular, and organ level. The gene for insulin was cloned in 1980 (2), and recombinant insulin has been available commercially since this time. Insulin therapy has recently taken a turn toward the use of insulin analogues instead of native human insulin formulations, and there are continued studies on alternative routes of delivery. Nevertheless, insulin remains one of the few drugs for which a suitable oral formulation has remained elusive.
The Discovery of Insulin
In 1869, a German medical student, Paul Langerhans, described a group of cells embedded within the acinar tissue of the pancreas that were dissimilar in structure to the rest of his histological preparation (1). It took another 30 years for secretory function to be ascribed to these “islets of Langerhans.” Once it became apparent that the “internal secretion” of these cells had a glucose-lowering effect, the race was on for its extraction. In 1922, Banting, Best, Collip, and Macleod isolated the glucose-lowering substance from beef pancreas and attempted to treat Leonard Thompson, a severely diabetic teenager (3). After a short initial setback due to impurities of the extract, Leonard Thompson began to respond and continued to take “insulin” for the rest of his life.
Type-1 Diabetes Mellitus
Type-1 diabetes mellitus (T1DM) is characterized by varying degrees of absolute (not relative) insulinopenia due to T-cell mediated autoimmune destruction of the pancreatic β cells. A decline in insulin-secreting capacity for months or years precedes the clinical manifestation of diabetes, which usually occurs once 80% of the β cells have been destroyed. It can present at any age, ranging from infancy to mid-adulthood. The incidence of T1DM has increased worldwide in all age groups (even in the very young) postulated to be due to environmental factors (4). In most countries, the highest incidence is found among children 10 to 14 years old (5) and 3 to 6 years of age. Both sexes are affected in equal parts. In the past, T1DM was thought to occur more frequently in Caucasians. However, newer reports suggest that, in certain regions in the United States, African American children may be affected as frequently as Caucasian children (6). Newly recognized cases are predominantly seen in the autumn and winter months, with many speculations as to the cause of this seasonal and long-term cyclical variation (7,8,9). Furthermore, the natural regional proclivity for T1DM to the northern hemisphere (such as Finland and Sweden) as opposed to the southern hemisphere (Peru and Venezuela) remains unexplained.
Common symptoms at the time of presentation include 2- to 3-week history of polyuria and polydipsia, weight loss, abdominal pain, anorexia, vomiting, and fatigue reflecting the metabolic effects of insulin deficiency. Especially in young children, a history of abdominal pain and vomiting is often misinterpreted as part of a viral syndrome. Therefore, routine urine testing of glucose and ketones are warranted in any child who presents with these complaints. Although the presence of glucosuria is a good screening tool, the following criteria have been established to confirm the diagnosis: a random serum glucose level of 200 mg per dL or more (11.1 mmol per L) must accompany symptoms suggestive of diabetes. Alternatively, a fasting serum glucose level of 126 mg per dL or more (7 mmol per L) can also establish the diagnosis. Glucosuria alone is not diagnostic of diabetes in children as it often accompanies a variety of renal tubular disorders. Glucosuria (with mild hyperglycemia) may also be stress induced during severe infection or steroid therapy. In these cases, hyperglycemia is transient and remits after convalescence. As soon as the diagnosis of diabetes is established, insulin therapy should be initiated promptly to prevent further metabolic decompensation and the development of diabetic ketoacidosis (DKA). After initiation of insulin therapy, there is a partial recovery of β-cell function. During this so-called honeymoon period, diabetes control is quite good and easy to achieve. The duration of the honeymoon period is variable, lasting from weeks up to 2 years, after which the insulin secretory capacity of the β cell declines permanently.
Physiologic Effect of Insulin
Although insulin has a profound effect on carbohydrates, it also exerts significant control over lipid, protein, and mineral metabolism. The most pronounced insulin effect is found at its three target tissues: liver, fat, and muscle.
The liver is a key organ in maintaining a steady state of serum glucose levels, tightly controlled by the insulin-to-glucagon ratio in the portal circulation. There is minimal direct insulin effect on the hepatocyte glucose transporter 2 (GLUT2); instead, insulin indirectly promotes glucose uptake into the liver by stimulating enzymes involved in glucose metabolism and storage. In a fasting state, low insulin levels enhance gluconeogenesis from glucose precursors, while the relative glucagon predominance stimulates glycogenolysis. Both of these processes are synergistic in achieving a steady fasting hepatic glucose production rate of 2 mg per kg per minute.
As soon as insulin levels rises postprandially, hepatic glucose production is suppressed and glycogenesis, glycolysis, and free fatty acid synthesis are enhanced. Once the liver is saturated with glycogen, any additional glucose, under the presence of insulin, is shunted to the synthesis of fatty acids. The fatty acids are esterified with α-glycerol phosphate to produce triglycerides, which are then transported as the very low-density lipoprotein (VLDL) fraction to the adipocyte.
In the adipose tissue, insulin enhances the activity of lipoprotein lipase, which is found in the capillary endothelium. Lipoprotein lipase hydrolyzes the triglycerides of the VLDL fraction to fatty acids and monoglycerides. Fatty acids are membrane permeable and can easily enter the adipocyte, where they are re-esterified to triglycerides. Insulin plays a key role in creating these fat stores by directly enhancing glucose uptake into the adipocyte through stimulating glucose transporter 4 (GLUT4), which facilitates glucose entry into the cell (see the “Insulin Receptor” section). Intracellular glucose in the adipocyte increases glycolysis, which increases the production of α-glycerol phosphate needed for fat esterification. Furthermore, insulin inhibits the breakdown of fat through inhibiting hormone-sensitive lipase, which induces lipolysis in the insulin-depleted state.
In the muscle, insulin’s main function is to facilitate glucose entry into the cell by binding to the insulin receptor, which then upregulates GLUT4. Once inside the muscle, glucose is used for immediate energy expenditure and for building up muscle glycogen stores. Insulin furthermore has an anabolic effect on the muscle, promoting positive nitrogen balance by enhancing amino-acid (especially branched chain amino acids) uptake, inhibiting protein breakdown, and to a lesser extent promoting protein synthesis. During fasting (low insulin state), glucose is mainly taken up by organs that do not depend on insulin for glucose transport, such as the brain.
Insulin also activates the sodium–potassium adenosine triphosphatase (ATPase) in many cells and therefore enhances potassium flux into the cell, even in the absence of glucose movement or pH changes.
Synthesis of Insulin
Insulin synthesis is confined to the pancreatic islet β cells. The β cells make up the bulk of the Langerhans islet and reside in the center of the islet closest to the blood supply. Glucagon-secreting α cells surround the β cells in a circular pattern. The outmost layer of the islet cell is formed by somatostatin- and gastrin-secreting δ cells. Islets containing α, β, and δ cells are mostly found in the anterior lobe, body, and tail of the pancreas. They are connected through gap junctions, a feature that has been proposed in facilitating the paracrine effect of insulin on glucagon and somatostatin. Polypeptide-producing F cells are also found in a portion of islet cells. They are restricted to those islets located in the posterior lobe of the pancreas head and are supplied by a separate vascular system. Even though the Langerhans islets are the most powerful regulators of glucose metabolism, they comprise less than 1% of the pancreatic organ mass. The average person has about 1 million islets varying in size from 50 to 300 μm in diameter.
Insulin originates in the form of a large precursor, preproinsulin, that is synthesized in the ribosome of the β cell’s rough endoplasmic reticulum. This 110-amino-acid peptide is encoded by a single gene located on chromosome 11 (10). Microsomal signal peptidases rapidly excise a terminal portion of the peptide chain, leaving 86-amino-acid proinsulin to be transported to the Golgi apparatus. There it is packaged into secretory vesicles, located close to the cell membrane. The 21 amino acids adjacent to the COOH terminus of the insulin protein are referred to as the α chain. The α chain is joined via a dipeptide bond to the 31-amino-acid c (connecting) peptide. Another dipeptide link connects the c-peptide chain further to the 30 amino acids of the β chain leading up to the protein’s NH2 terminus. The tertiary structure of insulin is created by the folding and oxidation of the proinsulin in which two disulfide bridges are created between the α and β chain and another disulfide bridge within the α chain. In the secretory granules, c-peptide is split off from proinsulin, resulting in equimolar release of insulin and c-peptide. The insulin secretion process involves fusion of the cell membrane and secretory granules with subsequent exocytosis of not only insulin and c-peptide but also small amounts of proinsulin. Although c-peptide has no insulin-like effect, proinsulin has about 7% to 8% of the biological activity of insulin (11). Unlike most protein hormones, the insulin structure is highly conserved among the species. Porcine insulin differs from human insulin only by one amino acid in the β chain and bovine insulin by three amino-acid residues.
Insulin Secretion
Although glucose is the strongest stimulator of insulin secretion, other sugars (e.g., mannose), certain amino acids (e.g., arginine, leucine), other hormones and neurotransmitters, and vagal nerve stimulation also modulate insulin secretion, keeping glucose concentrations in a narrow range.
In the rodent, glucose is transported into the β cell by a high-capacity (GLUT2) glucose transporter (12). GLUT2 is also the predominant glucose transporter in human pancreas and liver and is capable of bidirectional transport, allowing transport in either direction depending on the glucose concentration on each side of the membrane. Other GLUT isoforms (GLUT1 or GLUT3) that are ubiquitously present in most cells may also contribute to basal transport activity into the pancreas.
After uptake into the islet cell, glucose is phosphorylated to glucose-6-phosphate by glucokinase, the rate-limiting step of glucose metabolism in the islet cell (13). Glucokinase serves as the islets’ “glucose sensor” and its activity is tightly linked to glucose levels. Mutations in the glucokinase gene have been associated with defects in glucose sensing, necessitating higher glucose levels to stimulate insulin secretion. This defect is clinically referred to as MODY 2 (maturity onset diabetes of youth, form 2) leading to mild hyperglycemia (14,15).
Intracellular glucose metabolism raises ATP levels, which in turn close the potassium-dependent (K-) ATP channels in the β-cell membrane. The subsequent suppression of potassium (K) efflux leads to a progressive depolarization of the plasma membrane and the firing of action potentials. A drop in voltage follows, opening the voltage-sensitive L-type calcium channels for an influx of calcium ions (16). The rise of cytoplasmic calcium concentrations leads to a series of poorly defined reactions that culminate in the extrusion of insulin granules.
Each β cell contains thousands of K-ATP channels, which in fasting conditions are open, clamping the cell membrane potential at -70 mV. Sulphonylureas are hypoglycemic agents that are now known to modulate the K-ATP channel by attaching to a specific 145-kDa channel subunit named SUR (17). Sulphonylureas attach to the receptor and inhibit the K-ATP channel. The subsequent cascade of membrane depolarization and calcium influx then leads to an insulin secretory effect that is independent of serum glucose levels. Loss of function mutations in the gene encoding the β-cell potassium channel result in persistent hyperinsulinemic hypoglycemia of infancy (18,19). Gain of function mutations of the same gene results in certain types neonatal diabetes mellitus that can respond to sulphonylureas, and some patients can be taken off insulin (20,21,22).
Insulin is secreted directly into the portal vein and is immediately degraded by liver insulinases to approximately half of its original concentration. In serum, insulin levels rise to maximum levels 30 to 45 minutes after food ingestion and decline to basal levels by 2 to 3 hours postprandial. During prolonged fasting, both glucose and insulin levels will drop even further, but insulin levels will remain measurable between 2 and 5 μU per mL.
Insulin Receptor
Insulin action is mediated by the insulin receptor, a transmembrane glycoprotein present in virtually all vertebrates. Under healthy conditions, only a small proportion of the total available cell receptors are occupied to achieve maximal biological effect. High ambient insulin levels downregulate the insulin receptor by reducing the number of available insulin receptors. Furthermore, saturation of the receptor with insulin leads to “negative cooperativity,” a term used for reduced affinity for insulin in the adjacent receptor. The human insulin receptor was cloned in 1985 (23), and the gene maps to the short arm of chromosome 19 (24).
The structural components of the receptor are two extracellular α subunits that are linked to each other as well as to the two β subunits by disulfide bonds, forming a heterodimer. The α subunits are external to the cell membrane and contain the hormone-binding sites. The transmembrane-spanning β subunit has three domains: extracellular, transmembrane, and cytosolic. The cytosolic domain contains tyrosine protein kinase activity. The binding of insulin at the extracellular α subunit is transduced to the intracellular β subunits, leading to rapid onset of receptor autophosphorylation (25). Among the proteins phosphorylated are the insulin receptor substrates 1–3 (IRS-1–3). IRS-1 acts as a docking protein for other proteins such as phosphatidylinositol 3 (PI3)-kinase. The cellular products created by PI3-kinase in turn appear to activate the translocation of glucose transport proteins (GLUT4) to the cell surface, facilitating glucose uptake. GLUT4 is available in the cytoplasm of all cells that have insulin-sensitive glucose transport across the cell membrane. Once insulin binds to the receptor, GLUT4 migrates to the cell surface, allowing the cell to efficiently take up glucose.
After binding to the receptor, the receptor-insulin complex is internalized and processed to release of free insulin and recycling of the receptor back to the cell membrane. Whether the internalization of insulin permits any special action of insulin at the level of the nucleus remains a speculation, and insulin action beyond receptor activation is incompletely defined.
Insulin Signaling
Insulin’s binding to it’s receptor stimulates signal transduction pathway that culminate in GLUT4 translocation to the plasma membrane. GLUT4 is responsible for glucose uptake in all cells whose glucose transport across the cell membrane is insulin dependent. Once insulin binds to the receptor, two distinct intracellular signal transduction pathways lead to a migration of GLUT4 to the cell surface, allowing the cell to efficiently take up glucose.
The first pathway is often referred to as the IRS/phosphatidylinositol 3-kinase (PI3 K)/Akt pathway (26,27). In this pathway, IRS-1 is tyrosine phosphorylated by the insulin-stimulated tyrosine kinase activity of the insulin receptor. This allows the regulatory unit (p85) of PI3 K to dock onto the IRS-1 which in turn activates PI3 K. This leads to a generation of phosphatidylinositol 3,4,5-triphosphate (PIP3) which in turn activates the Ser/Thr kinases Akt and atypical protein kinase C (aPKC). These kinases are essential in the process of GLUT4 translocation to the cell surface, facilitating glucose uptake. The second pathway is also referred to as the Cbl–CAP pathway (28,29). Under the presence of the adapter protein CAP, the Cbl proto-oncoprotein is tyrosine phosphorylated (28). The CAP–Cbl complex then dissociates from the insulin receptor and move to a lipid raft subdomain of the plasma membrane for a second route of GLUT4 translocation (30).
Insulin Treatment
Although normoglycemia is a fairly concise treatment goal in diabetes management, the clinical use of insulin is remarkably complex. With so many nutritional, hormonal, and lifestyle variables affecting glucose homeostasis, there is rarely a predictable treatment algorithm that applies to all patients, especially in children and adolescents with T1DM. Even in a single patient, treatment regiments remain under constant scrutiny warranting regular reassessments and fine-tuning. Therefore, insulin regimens are never absolute, but loose guidelines that mold with the patient throughout life.
In general, insulin requirements in a patient with newly diagnosed type-1 diabetes are calculated based on the age, pubertal status, and the presence of ketonuria. Young children (toddlers) who do not present with ketoacidosis may only require 0.3 units per kg per day. The average prepubertal child will require 0.5 to 0.75 units per kg per day, possibly more in the presence of ketosis. Pubertal children will be more insulin resistant due to their higher physiologic levels of growth hormone and usually require 1 to 1.5 units per kg per day. Within 1 to 3 weeks of commencing therapy, insulin requirements usually decrease drastically as partial remission (honeymoon period) occurs. To maximize the duration of remission, it is recommended that a twice-daily insulin regimen is maintained (even at a low dose of 0.2 units per kg per day), as long as episodes of hypoglycemia do not ensue.
Animal and Human Insulin Preparations
Today pure animal insulins are obsolete in the management of diabetes mellitus. However, the different animal preparations with their various pharmacodynamics have become the prototype after which synthetic human insulins are modeled. They are therefore of exemplifying interest.
Shortly after the discovery of insulin, pork and beef insulin products became the treatment of choice for several decades. Early on in the commercial production, trace quantities of zinc were added to produce “regular” insulin, a more stable (crystalline zinc) insulin (31). The duration of this regular insulin was on average 5 to 8 hours, often too short for overnight glucose control. Protamine, a basic fish (trout) protein, was added to slow down the absorption from the subcutis by decreasing the solubility at physiologic pH (32). Combining both zinc and protamine further extended absorption time, leading to insulin action in excess of 24 hours (33). In 1946, the Hagedorn Laboratories produced a protamine zinc insulin in which protamine was added in stoichiometric proportions to insulin, thereby reducing excess free protamine in solution. Hagedorn coined the name “isophane” (Greek: equal appearance) for the long-acting insulin, but the name did not stick and it became known as NPH (neutral protamine Hagedorn) insulin. Another method to prolongate insulin action without adding a protein was the creation of a zinc–insulin complex in the presence of large amounts of zinc and acetate buffer. Under careful pH adjustments, this insulin could be produced in a completely crystalline form (ultralente) or as an amorphous precipitate (semilente). The combination of these two zinc-acetate insulins (70% ultralente and 30% semilente) created lente insulin, with a similar duration to NPH. One of the major limitations of these animal insulins was their immunogenic potential, stemming from contamination with pancreatic extract impurities, such as animal c-peptide, proinsulin, glucagon, somatostatin, and pancreatic polypeptide (34). The foreign amino-acid sequence itself was also proposed to contribute to immunogenicity, leading to insulin resistance (35). To avoid the effect of a foreign protein sequence, a semisynthetic insulin was developed in which the human amino-acid sequence was created by switching out the differentiating amino acid in porcine insulin (36). To date, this is the only porcine-based insulin still available commercially.
With the advent of recombinant DNA technology, semisynthetic human insulins were soon joined by the production of completely synthetic human insulin in the 1980s. The initial process entailed separate production of the α and β chains from Escherichia coli with biochemical combination of the two chains to yield an intact molecule (37). In 1986, human insulin could be produced in its entirety from synthetic proinsulin. This method includes the insertion of a synthetic proinsulin into the genome of baker’s yeast with subsequent enzymatic yield of human insulin and c-peptide. This technique allows for natural posttranslational folding of the peptide and ensures the appropriate three-dimensional structure of the molecule (38,39).
Human insulins are also marketed as regular (short acting), NPH and lente (intermediate acting), and ultralente (long acting) with identical formulations as their animal counterparts in respect to content of auxiliary substances. They have very similar biological potency to animal insulin when given intravenously (40,41). However, subcutaneous administration of intermediate- or long-acting human insulin has a shorter time-action profile than animal insulin.
Insulin Regimens
The most common initial insulin regimen after diagnosis is a twice-daily injection of a mixture of human intermediate-acting (NPH) or long-acting (detemir, glargine) insulin and short-acting insulin (regular insulin) or rapid-acting insulin (insulin lispro, insulin aspart, insulin glulisine). In general, when patients are on a NPH or detemir insulin with a rapid-acting insulin regimen, two-third of the total dose is given before breakfast and one-third before dinner. The prebreakfast and predinner doses are composed of two-third intermediate-acting insulin and one-third short-acting insulin and given as a single (mixed) injection. Glargine is considered a 24-hour insulin, with some exceptions; therefore, patients receive a once a day injection of glargine with rapid-acting insulin injection for each meal.
The two-daily insulin injection regimen could be sufficient during the honeymoon period due to the fact that the endogenous insulin secretion provides much of the overnight basal insulin requirements, leading to normal fasting blood glucose values. Thus, increased and more labile prebreakfast glucose levels herald the loss of residual β-cell function often necessitating a third injection. To contain rising morning blood glucose values, the predinner dose can be separated into an injection of short-acting insulin before dinner and long-acting insulin before bedtime, thereby delaying peak action to the early morning hours. Although theoretically sound, the challenge remains to achieve normoglycemia in the morning, while avoiding nocturnal hypoglycemia during the nighttime fast. The physiologic basis for this battle with morning hyperglycemia is the dawn phenomenon, an early morning surge of counter-regulatory hormones, mainly growth hormone and cortisol. Dawn phenomenon must be differentiated from rebound hyperglycemia (Somogyi effect) that results from a counter-regulatory hormone rise in response to an untoward nocturnal hypoglycemia. Most diabetes care providers have no hesitations switching to a variety of three-injection regimens, trying to match varying peak actions with the lifestyle of the patient and his or her family. Prefilled, disposable pen devices of one type of insulin have made it easier for patients to accept split dose regimens or extra injections before lunch or before an afternoon snack. Various jet (transcutaneous pressure) injectors have also become available commercially over the last decade. Theoretically, they are indicated for children with needle phobia and are deemed to have the therapeutic advantage of increased accuracy and better subcutaneous dispersion with subsequent faster absorption. Because they are cumbersome to use and often traumatize the skin (causing greater discomfort), they have not penetrated the pediatric market very well. Disposable insulin pens with ultrafine short needles are by far the more popular alternative for a single insulin injection.
To gain better control, a third type of insulin, such as ultralente, was sometimes added to the morning or evening injection mixture to cover for waning NPH levels in the late afternoon and early morning. However, with the recent advent of the 24-hour peakless insulin glargine analogue, such regimens became a managing style of the past. Insulin glargine has a flatter and longer time-activity profile than NPH or ultralente and therefore more closely mimics the basal insulin activity of the pancreas. The only disadvantage of insulin glargine in multiple injection regimens is that it cannot be mixed with other insulins, requiring a separate injection. Unlike other long-acting insulins that are cloudy in appearance, insulin glargine is clear like the short-acting insulins. There is concern that it would be easy to confuse the two types of insulin, leading to significant dosing and administration errors. However, the fact that insulin glargine is fully suspended in solution may decrease technical errors, as it does not require resuspension prior to administration. Disposable pens with premixed-intermediate (NPH) and short-acting (insulin lispro or regular) insulin are also available, but they are rigid in their composition and allow little flexibility in fine-tuning glycemic control. They are usually used in patients whose compliance with insulin administration is at stake and the simplest dosing regimen is maintained to avoid DKA. Sometimes, these premixed preparations are indicated if it is an impossible feat for the patient or caregiver to mix insulin, resulting in major dosing errors.
When interpreting blood glucose readings, a number of factors that affect injection therapy must be considered prior to making an actual insulin dose adjustment. First and foremost, compliance and administration technique must be considered. From a psychosocial aspect, children and adolescents will often painstakingly cover up missed injections. A review of skills is equally important in uncovering technical slips, which can lead to poor glycemic control. For example, the long-acting insulins such as lente and NPH need to be resuspended prior to injection for concentration constancy between injections. Omitting this crucial step leads to great variability in glycemic control. Furthermore, the presence of hypertrophied injection sites, which results from local growth factor effect of repeated insulin injections into the same site, can slow down or decrease absorption. The site location is another factor that comes into the equation when fine-tuning insulin regimens. Insulin is absorbed most rapidly from the subcutaneous abdomen and less rapidly from the arms. Absorption is most delayed from the subcutaneous tissue in the legs. The depth of injection, the ambient temperature or the state of perfusion at the injection site can also affect absorption.
An alternative to multiple-daily injection that has revolutionized diabetes management is continuous subcutaneous insulin infusion (CSII) therapy, also referred to as insulin pump therapy. For many families, this is the preferred choice for achieving the goals of intensive therapy with increased lifestyle flexibility.
Insulin Analogues
Insulin analogues are modified human insulin molecules with altered pharmacodynamic and physicochemical properties. Using molecular technology, they were specifically designed to create a molecule to mimic endogenous insulin action. Despite the challenges with subcutaneous absorption particularly for rapid insulin analogues, both rapid- and long-acting new insulin analogues have made achieving target glycemic control with less hypoglycemia a possibility.
Rapid Insulin Analogues
New rapid insulin analogues (lispro, aspart, glulisine) reduced postprandial glucose excursions as compared to regular insulin with their earlier absorption and action profiles. The convenience of taking the injection 15 minutes before or even with the meal as opposed to 30 to 40 minutes prior to the meal also led to better compliance with therapy. Faster onset of action, earlier peak activity, and higher peak concentrations of rapid-acting analogues are particularly beneficial in overcoming problems associated with insulin resistance of puberty.
The first insulin analogue in this group did not quite make it to clinical use because of one important side effect. Asp (B10) insulin was engineered by replacing the naturally occurring histidine with aspartic acid on residue 10 of the insulin β chain and was found to be absorbed twice as fast as regular insulin (42). However, this simple amino-acid exchange altered the three-dimensional structure of insulin, leading to increased affinity of this molecule to the structurally related insulin-like growth factor (IGF)-1 receptor. Furthermore, Asp (B10) insulin demonstrated extended activation of the insulin receptor with decreased dissociation and prolonged stimulation of cellular processing (43,44). This mitogenic risk was confirmed in Sprague-Dawley rats that developed mammary tumors after supraphysiologic doses of this analogue. Further human application of this analogue has since then been halted.
In 1996, the first rapid-acting insulin analogue, insulin lispro, was FDA approved for clinical use. Insulin lispro received its name from its chemical structure in which the amino-acid lysine at position 29 of the β chain was exchanged with the position 28 proline of the same chain. This amino-acid reversal decreases the tendency to self-associate, leading to faster absorption, higher peak levels, and shorter duration of action (45). Studies comparing regular insulin and insulin lispro demonstrated no difference in affinity to the insulin receptor. There is a higher affinity to the IGF-1 receptor, but this did not cause a difference in growth-stimulating activity when compared to regular insulin (46). Clinical trials have demonstrated that insulin lispro acts within 15 minutes, peaks at approximately 1 hour, and lasts 2 to 4 hours after subcutaneous injection (45,47). Insulin lispro is stable in CSII systems and has been shown to improve postprandial glucose levels when CSII therapy was compared using regular human insulin (48,49,50). There is no difference in the frequency of catheter occlusion or other site-related problems with insulin lispro as compared to buffered regular insulin (49,50). However, there were recent reports of a few patients who developed marked lipoatrophy while using insulin lispro during CSII (51,52). The basis of this lipoatrophy is unclear but was resolved with use of regular human insulin. In the past, lipoatrophy was seen with the use of less purified insulins and was assumed to have an immunologic basis. Contrasting are the reports of similar immunogenicity between insulin lispro and recombinant human regular insulin (53). Also, insulin lispro has been a successful substitute for human regular insulin in several cases of presumed immunogenic insulin resistance (54,55). A concern for the use of insulin lispro (or any other rapid-acting insulin) in CSII is its short duration of action, possibly increasing the risk of DKA in cases of mechanical malfunction or catheter occlusion (56).
Another synthetic insulin analogue, insulin aspart, was approved for clinical use in the United States in June 2000. In this molecule, proline at position 28 of the β chain is substituted with negatively charged aspartic acid to reduce self-association and therefore increase rate of absorption (57). Preclinical studies have shown that the insulin aspart has the same insulin receptor and IGF-1 receptor interaction kinetics as human insulin, diminishing concerns about the mitogenic potential of insulin aspart. Similar to insulin lispro insulin, insulin aspart was conceptualized as a meal-time insulin. It is absorbed more rapidly than human regular insulin, reaches higher maximal concentrations postprandially, and has a shorter duration of action (57,58,59). In a multicenter study, the risk of postprandial hypoglycemia requiring third-party intervention was reduced significantly without leading to deterioration of late postprandial blood glucose levels when insulin aspart was compared to regular insulin (60).
The most recent addition to the rapid-acting insulin analogue group is insulin glulisine. It has similar pharmacokinetic, pharmacodynamic, and safety profile as compared to other rapid-acting insulin analogues. The neutral asparagine is exchanged for basic lysine at position 3 and acidic glutamic exchanged for basic lysine at position 29 as compared to human insulin β chain leading to improved solubility (61). Glulisine is formulated without the addition of zinc. It also has a different effect on activation of IRS1 and IRS2 with decreased activation of IRS1 shown by animal studies suggesting favorable effects on decreasing apoptosis (62,63). It does not appear to accumulate in patients with renal impairment (64). Insulin glulisine is comparable to other rapid-acting analogues with respect to glycemic control, and its somewhat faster absorption rate has questionable clinical significance (65). Postmeal administration of glulisine was associated with a slight reduction of weight (-0.3 kg) while premeal administration was associated with minor weight gain (+0.3 kg) (62).
After the introduction of insulin, patients experienced cutaneous reactions directly related to insulin. These immunologic reactions and skin alterations were stemming from foreign proteins from animal insulins acting as allergens. Later on, preservatives such as metacresol or agents to prolong action such as zinc protamine were reported to be the culprit of these reaction. Prevalence of hypersensitivity reactions substantially declined from 50% to 60% (66,67). Insulin therapy related lipodystrophies are still not obsolete. This group encompasses lipohypertrophy and lipoatrophy. Lipohypertrophy is a result of the insulin anabolic effect, promoting adipose tissue differentiation and growth (68). Lipoatrophy is a relatively uncommon allergic reaction mainly due to the impure insulin molecule. Fat atrophy is the result of lysosomal enzyme activity triggered by an immune-mediated inflammation (69). Lipoatrophy is not merely a cosmetic issue since the absorption of insulin from these sites is erratic (70). Local steroid injections, alternative methods of insulin delivery have been proposed for the management of lipoatrophic sites and achieved some success (71,72,73).
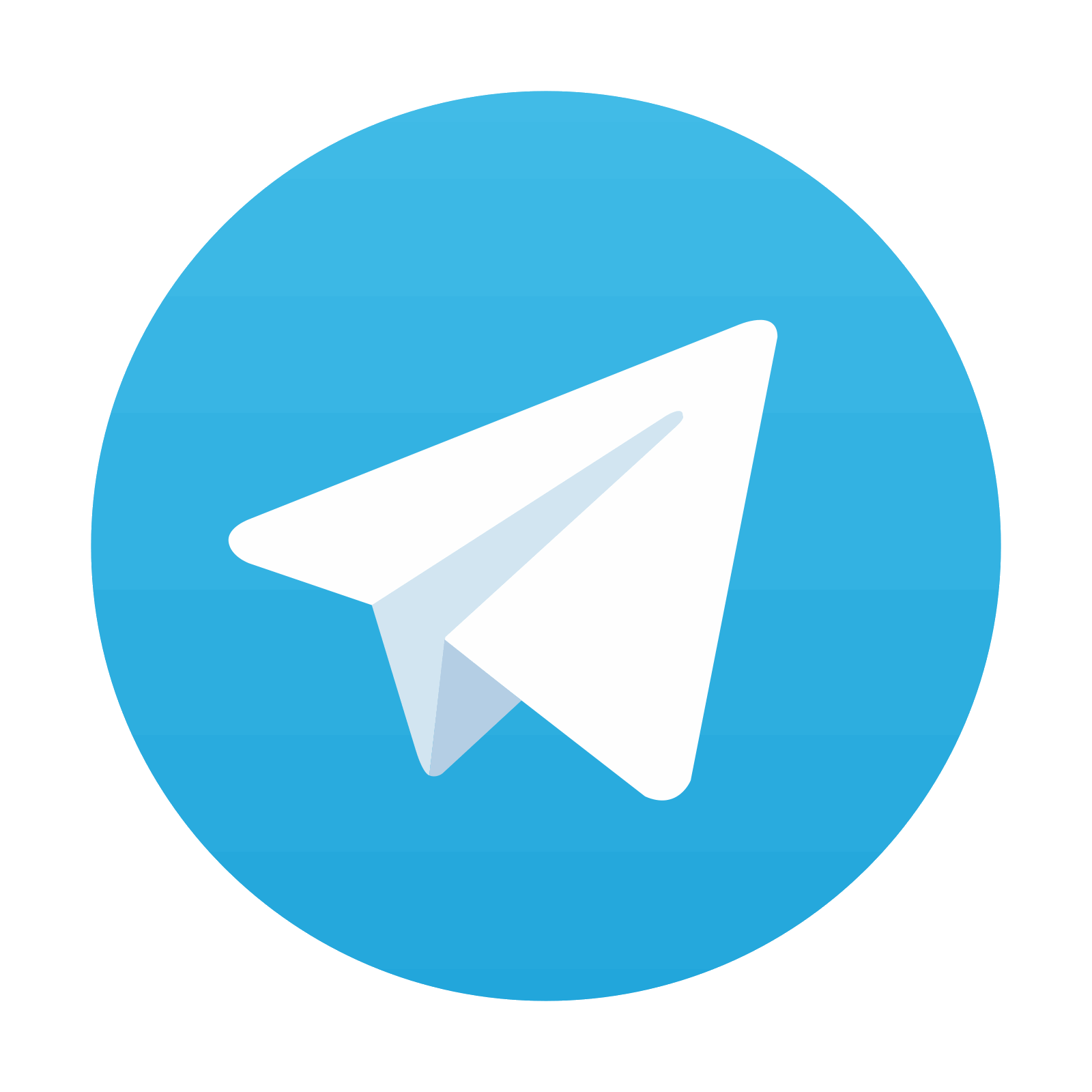
Stay updated, free articles. Join our Telegram channel
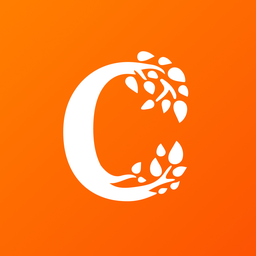
Full access? Get Clinical Tree
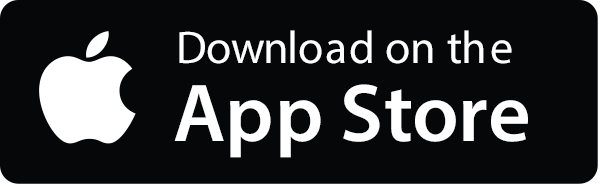
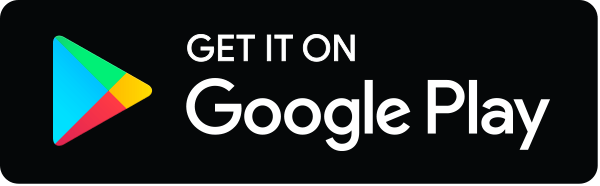