The development of biomaterials used in the manufacturing of temporary or permanent implantable prosthetic devices has been one of the greatest advances in modern medicine. These devices have become an integral and important part of the current practice of medicine and have improved considerably the lives and health of countless patients. In the United States, the number and types of permanent prosthetic devices implanted to replace diseased or damaged body parts has increased substantially during the past several decades. An estimated 3 million or more people in the United States currently have some type of long-term biomedical implant. Box 74.1 lists implantable prosthetic devices discussed in this chapter.
-
Central nervous system shunts
-
Intracranial pressure monitors
-
Intrathecal pump infusion
-
Orthopedic prostheses (artificial hip, knee, and other joints; screws, pins, plates, and rods)
-
Intracardiac pacemakers and defibrillators
-
Left ventricular assist devices
-
Extracorporeal membrane oxygenation circuits
-
Ocular prostheses (artificial globes, intraocular lenses, ocular explants)
-
Cochlear implant devices
-
Tissue expander devices
One of the major medical complications associated with the use of implantable prosthetic devices is infection, which may result in serious tissue destruction and dysfunction of the prosthetic device or in local and systemic consequences that may be life threatening. In most cases, these infections are very difficult to cure with antimicrobial agents alone, and removal of the device usually is required for resolution of the infection.
Interaction of the Host With a Prosthetic Device
The prosthetic devices currently in use are composed of a variety of biomaterials, including cobalt-chromium-molybdenum alloy, titanium alloy, and complex polymers such as polytetrafluoroethylene, silicone, and polyethylene, which in general are chosen for their inert, nonreactive, and nontoxic qualities. The interplay of both implant and host factors determines the risk for acquiring and the severity of infection. The human body has numerous well-developed defense mechanisms to protect it against possible invasion by various microorganisms. Such mechanisms include cellular and humoral immune systems, anatomic barriers, and an elaborate network of cells that phagocytize and destroy invading organisms. The presence of a foreign body may compromise one or more of these defenses and elicit a complex acute or chronic inflammatory response (or both) from the host. Many of these devices breach cutaneous and mucosal barriers, thereby creating a direct route by which bacteria and fungi may invade. In addition, implanted devices may alter the local immunity of the host directly or indirectly.
Shortly after being implanted, hydrophobic polymeric materials such as polyethylene, Dacron, polydimethylsiloxane, and polyether urethanes become coated with a layer of host proteins such as plasma and interstitial fluid proteins (i.e., fibronectin, albumin, laminin, collagen, immunoglobulin G, fibrinogen) that bind to and are absorbed readily into the surface of the implant. This protein layer (especially fibrinogen) has a major influence on the body’s response to and the biocompatibility of the implant. The presence of fibrinogen attracts a large number of phagocytic cells (i.e., neutrophils, monocytes, and macrophages) to the implant; these cells interact with the implant surface and initiate an acute inflammatory response. Some of these host proteins also serve as a receptor for various colonizing microorganisms. Collagen, laminin, and fibrinogen have been reported to play a role in adherence of bacteria, and fibronectin has been found to be the major receptor for gram-positive cocci, especially Staphylococcus aureus.
Chronic inflammatory responses, also known as foreign body reactions, are seen around many types of biomaterial implants and arise from interactions among the protein-coated surfaces of the implant and host tissues and adhering phagocytic cells such as macrophages and foreign body giant cells. These interactions may result in degradation and damage to the implant from the continuous generation of toxic catabolites and release of inflammatory mediators such as hydrolases, activated complement components, tumor necrosis factor (TNF), interleukins, prostaglandins, coagulation factors, and plasminogen activator by the phagocytic cells.
Interaction of Microorganisms With a Prosthetic Device
Once a prosthetic device is implanted, the surface of the device provides a potential area for adherence and multiplication of bacteria. Adherence is a complex process that involves electrostatic attachment of the bacteria to the surface of the implant, bacterial mechanisms that function specifically in attachment, and host-derived substances that coat the prosthetic device and serve as receptors for various bacteria. Bacteria arrive at the surface of the implant by many different routes; they may be inoculated at the time of implantation, the patient may have episodes of transient bacteremia, the device may be exposed by local trauma or infection, or the device may be implanted within an area in which the organism is part of the normal flora.
Adherence of bacteria to the surface of an implant is influenced by numerous different factors, including the material used to make the device, the source of the device material (adherence is greater with synthetic material than with biomaterial), the surface of the device (i.e., irregular more than regular, textured more than smooth, hydrophobic more than hydrophilic), and the shape of the device. Cell surface molecules or structures known as adhesins also play a role in adherence by attaching or binding an organism to specific receptors on implant surfaces; different bacteria use different adhesins to attach to and colonize medical implants. For example, Staphylococcus epidermidis uses proteinaceous autolysin and capsular polysaccharide intercellular adhesin for initial adherence to the implant surface and for adherence of bacteria to each other. These bacteria also produce a biofilm that increases cell-to-cell association and allows accumulation of bacteria. Streptococcus pyogenes uses lipoteichoic acid as its adhesin, whereas S. aureus uses both lipoteichoic acid and host-tissue ligands (e.g., fibronectin, fibrinogen, and collagen) for adherence. Binding of S. aureus to host-tissue ligands is mediated by genetically defined microbial surface proteins known as microbial surface components recognizing adhesive matrix molecules (MSCRAMM). Escherichia coli and other bacteria use fimbriae as an adhesin to mediate binding to receptors on the surfaces of target cells.
Bacteria also can protect themselves from host defenses by synthesizing and excreting numerous complex polysaccharides, known as glycocalyces, that function either as part of the bacterial capsule or as the slime layer. This slime layer is known to play a major role in keeping an organism attached to an implant surface by coalescing with the polysaccharides of other bacteria and with host products to produce a thick, adherent, and somewhat impenetrable biofilm. The biofilm functions by trapping nutrients and protecting the organism from phagocytosis, antimicrobial agents, and competing microflora. It also plays a role in inhibiting the response to chemotactic stimuli, increases both N -formyl-methionyl-leucyl-phenylalanine (FMLP)-induced superoxide generation and release of specific granules, and impairs natural killer cell function while also altering the composition of T-lymphocyte cell subpopulations. Therefore the biofilm aids bacteria in evading host cellular and humoral defense mechanisms and thereby allows the organism to colonize and infect an implanted device effectively.
Substantial progress has been made in our understanding of the pathogenesis, prevention, and treatment of foreign body infections; this increased knowledge has resulted in a dramatic decrease in the morbidity associated with these infections, as well as subsequent improvement in the patient’s quality of life. The following sections discuss specific device-related infections and the suggested treatment and management of these infections.
Tissue Expanders
The use of soft tissue expansion in reconstructive surgery was reported in the literature first in 1957, and since that time it has been used widely for the correction of multiple problems in plastic and reconstructive surgery in the adult and pediatric populations. These expanders consist of an alloplastic prosthesis with a filling port that is implanted into a subcutaneous pocket. The expander is filled with saline through the filling port at various intervals to create adequate expansion of the skin. Subsequent improvements of this technique led to the development of osmotic tissue expanders. The Osmed osmotic tissue expander is made of an osmotically active hydrogel (vinyl pyrrolidone and methyl methacrylate) in a silicone construct. When implanted, water is drawn from the surrounding tissues into the device, which can spontaneously expand up to 10 times its original volume. In children, tissue expansion is used most commonly to provide coverage for skin defects caused by burns, trauma, hemangiomas, and other congenital deformities.
The most common complication of tissue expansion is infection of the subcutaneous expander pocket, usually with skin organisms introduced during insertion of the expander. Cellulitis of the overlying skin and hematogenous or lymphatic seeding of the expander pocket are seen as well but occur much less frequently. In several pediatric case series, the infection rate ranged from 3.4% to 11%. S. aureus, S. epidermidis, and group A streptococci are the microorganisms recovered most commonly from these infections. Less commonly, nontypeable Haemophilus influenzae, Pseudomonas aeruginosa, E. coli, and Actinomyces spp. have been isolated from infections of expander pockets.
In cases of infection of subcutaneous pockets, treatment consists of removal of the tissue expander, debridement and drainage of the subcutaneous pocket, and administration of intravenous antibiotic therapy tailored to the organism isolated. For cellulitis of the overlying skin of an expander pocket, treatment with intravenous antibiotics but without removal of the tissue expander has been shown to be successful.
The most common empirical antimicrobial therapeutic regimen consists of a first-generation cephalosporin or an extended-spectrum, penicillinase-resistant penicillin. For all of the infections of prosthetic devices discussed in the chapter, empirical antistaphylococcal coverage should include agents effective against community strains of methicillin-resistant S. aureus (MRSA) if warranted by their frequency in the area.
Therapy is tailored once the organism has been identified and its antibiotic susceptibility has been determined.
Cochlear Implants
During the past several decades, cochlear implantation has emerged as one of the best methods of providing auditory rehabilitation for the profoundly deaf (congenital or acquired). The goal of this surgery in young children is to provide hearing that is adequate to facilitate the development of receptive and expressive language. The surgical technique involves the creation of a C-shaped flap in the postauricular and parietal-occipital scalp skin areas, elevation of the flap, implantation of a multichannel prosthesis, and insertion of an electrode array into the cochlea through openings drilled into the temporal bone.
The most common infectious complications associated with these implants are cellulitis of the overlying skin flap, acute mastoiditis, meningitis, otitis media, and delayed cochlear implant infections leading to extrusion of the implant. *
* References .
Rates of infection range from 0.3% to 0.5% for meningitis, 2% to 12% for cellulitis of the skin flap and delayed cochlear implant infections, and to 36% for otitis media. The reported cases of meningitis have occurred in association with leakage of cerebrospinal fluid (CSF) in persons with a malformed cochlea who undergo cochlear implantation, as a consequence of intracranial spread of a developing middle ear infection along the electrode pathway, or via pneumococcal bacteremia with hematogenous seeding of the cochlea—for example, at a site of tissue necrosis related to the electrode or positioner with contiguous spread to the CSF and meninges. In June 2002, the U.S. Food and Drug Administration (FDA) received numerous reports of bacterial meningitis in children with cochlear implants who were younger than 6 years when they received the implants. The most common causative organism identified was Streptococcus pneumoniae, followed by nontypeable and type b H. influenzae . Other less common organisms that have been reported include S. pyogenes, Acinetobacter baumannii, E. coli , and Enterococcus spp. The incidence of pneumococcal meningitis in this group of patients was calculated to be 138.2 cases per 100,000 person-years—more than 30 times the incidence in the same-aged cohort in the general population. This increased incidence of meningitis was found to be associated strongly with the use of a cochlear implant with a positioner (a wedge-shaped insert that facilitates transmission of the electrical signal by pushing the electrode against the medial wall of the cochlea) in conjunction with the presence of radiographic evidence of a malformation of the inner ear and leakage of CSF. Cochlear implants with a positioner were voluntarily recalled in the United States in July 2002, although removal of existing implants containing a positioner was not recommended; use of appropriate vaccination against S. pneumoniae and type b H. influenzae was strongly recommended. On February 24, 2010, a 13-valent pneumococcal conjugate vaccine (PCV13) was licensed by the FDA. This vaccine contains polysaccharides of the seven serotypes in PCV7 and polysaccharides from six additional serotypes. This vaccine replaces PCV7 for all scheduled doses of PCV7 in infants. A supplemental dose of PCV13 is recommended for children 14 months through 18 years of age with a cochlear implant. Children with cochlear implants may have a higher risk for middle ear infections because of several factors, including the naturally high incidence of acute otitis media in this population, the presence of a foreign body in the area of the infection, and the potential for spread of the infection into the cochlea along the electrode pathway. In a study of 50 children who received cochlear implants between 1991 and 1995, researchers found that children prone to the development of otitis media before undergoing implantation were at higher risk for developing postimplantation acute otitis media but responded well to routine oral antimicrobial therapy. The overall prevalence and the severity of acute otitis media were not found to be increased in children with cochlear implants.In cases of cellulitis of the skin flap, intravenous antimicrobial therapy should include empirical broad-spectrum antimicrobial agents effective against community strains of MRSA, methicillin-susceptible strains of S. aureus, and group A streptococci. Empirical antimicrobial therapy for meningitis usually consists of vancomycin and a third-generation cephalosporin that is tailored to the organism isolated. An exception is for children with the onset of meningitis during the first 2 weeks after cochlear implantation; in these instances, causal bacteria may include a broader range of pathogens, including gram-negative bacilli and gram-positive bacteria. Selection of a combination of antimicrobial agents that provides broader-spectrum coverage against gram-negative bacilli should be chosen. For delayed development of cochlear implant infections, therapy consists of removal of the implant and administration of intravenous antibiotics.
Ocular Prostheses
The ocular prosthetic devices include artificial globes used primarily for cosmetic purposes, orbital implants, ocular explants, intraocular lenses, and contact lenses.
Orbital Implants
Orbital implants are made of hydroxyapatite or porous polyethylene and frequently are used in orbital reconstruction after enucleation or evisceration surgery. Infection of these implants is a rare event, with only a handful of cases reported in the literature. Patients most commonly report anophthalmic socket pain or discomfort, pain with eye movement, and excessive conjunctival edema and irritation while wearing an artificial globe. Papillary conjunctivitis of the socket with exudate and sometimes dehiscence of the overlying conjunctiva also may be seen. Infection may develop months to years after placement of the implant, and severity ranges from cellulitis to the development of an abscess around the implant itself, with implant extrusion. In a retrospective clinical case series of 103 patients who underwent hydroxyapatite implant insertion at one institution, patients who had a freestanding polycarbonate peg placed to enhance the motility of their ocular prostheses had a significantly higher rate of infection than those patients with nonpegged hydroxyapatite implants, at 42.9% versus 19.5%.
Radiographic studies that can aid in the detection of these types of infection include technetium 99m–labeled leukocyte scintigraphy, which is most useful in detecting early low-grade graft infection, and computed tomography (CT) and magnetic resonance imaging (MRI), which are useful later in the course of the infection to detect the presence of abscesses and structural tissue changes. Gram-positive cocci, primarily S. aureus, and coagulase-negative staphylococci are the organisms associated most commonly with these infections; however, H. influenzae, S. pneumoniae, α-hemolytic streptococci, Capnocytophaga, Pseudomonas, and Aspergillus fumigatus have been cultured as well. To cure the infection effectively, treatment involves both removal of the implant and institution of topical and parenteral antibiotic therapy directed against the organism isolated. Empirical therapy directed against gram-positive organisms may be started initially until the results of culture and sensitivity testing are available. This should include antimicrobial agents effective against community strains of MRSA. In addition, other common empirical regimens include a first- or second-generation cephalosporin or an extended-spectrum, penicillinase-resistant penicillin.
Intraocular Lenses
Insertion of polymethyl methacrylate (PMMA) intraocular lenses at the time of removal of a cataract is the standard surgical therapy for this disorder. Even though the rate of postoperative infection of intraoperative lenses is low (0.10–0.30%), the infection usually is serious and results in endophthalmitis and permanent loss of vision. The predisposing factor for infection is bacterial adhesion to intraocular lenses during insertion. After adhesion is accomplished, the bacteria replicate, congregate, and form multiple layers of microcolonies that represent a biofilm in which the bacteria are embedded in a layer of slime. The major pathogens associated with this infection are S. aureus and S. epidermidis , which account for 90% of all isolates, although α-hemolytic Streptococcus spp. especially Streptococcus pneumoniae, gram-negative bacilli, Pseudomonas, fungi, Chlamydia trachomatis , Nocardia spp., and rapidly growing mycobacteria are isolated on occasion. Intraocular lens–associated endophthalmitis is a serious infection that is difficult to diagnose and treat. In a few cases, use of topical and systemic antibiotics alone has been successful in eradicating the infection, but in most cases surgical debridement and systemic antibiotics are required for cure. Empirical therapy for these infections consists of an extended-spectrum, penicillinase-resistant penicillin, a second-generation cephalosporin, or, in some instances, clindamycin or vancomycin. In cases of fungal endophthalmitis, treatment with the later-generation azoles alone and in combination with an echinocandin has been shown to have clinical success. In rapidly growing mycobacterial endophthalmitis combinations of topical and intravenous antibiotics to which the organism is susceptible have been used for treatment.
Contact Lenses
Primarily three different types of contact lenses are available. Hard lenses are made of PMMA, a substance that is impermeable to water and gas. This type of lens is designed to be worn only during waking hours because it limits oxygen flow to the cornea to that present in tears. Gas-permeable hard lenses are composed of silicone, cellulose acetate butyrate, or PMMA-silicone copolymers; they allow gas but not fluid to pass through the lens. Hydrophilic or soft lenses are made of a cross-linked hydrogel polymer or copolymer and consist of 38% to 85% water by weight; these lenses are permeable to both gas and water and allow the user to wear them continuously. However, for all these types of lenses, infection may result in damage to the corneal epithelium.
The two main infections that occur in association with contact lenses are conjunctivitis and keratitis. The causative bacteria seen most commonly with these infections are S. aureus, streptococci, Pseudomonas spp. (found in improperly stored cleansing solutions), and fungi (including Candida spp., Alternaria, Paecilomyces, Fusarium, Curvularia, and other filamentous fungi, and Acremonium ). Improper cleaning of soft or hydrophilic lenses may cause them to become a source of infection when bacteria penetrate the lens matrix. Risk factors for fungal keratitis include use of contaminated contact lens solution, trauma, contact lens wear, ocular surface disease, systemic diseases affecting the immune system, diabetes, use of systemic steroids, and recent chemotherapy. Fungal keratitis frequently is a severe disease in which diagnosis may be difficult, response to medical therapy is generally slow, and the clinical outcome may be poor. Corneal perforation is 5 to 6 times more likely with fungal keratitis than in bacterial keratitis. In a retrospective multicenter case series of 733 cases of fungal keratitis that looked at the epidemiology and microbiologic characteristics of fungal keratitis at tertiary eye care centers in the United States, it was found that filamentous fungi were more commonly found as the cause of keratitis among refractive contact lens wearers whereas yeasts, especially Candida spp., were the most common causative organisms in patients with ocular surface disease and ocular trauma. Treatment of keratitis usually consists of removal of the lens and application of topical antibiotics or antifungals. The most commonly used topical antifungal regimen is a combination of natamycin 5% and amphotericin B 0.15%. Twenty-five percent of patients also may require systemic antifungal therapy in addition to the topical therapy. In fungal keratitis, 16% to 26% of patients require surgical intervention with therapeutic keratoplasty in addition to medical therapy. To prevent the development of infections associated with contact lenses, users should adhere strictly to the manufacturer’s suggested guidelines for wearing and cleaning the lenses.
A rare and often devastating infection associated with the use of contact lenses is infection with the fresh-water protozoan Acanthamoeba. This organism contaminates the lens when sterility of the cleansing solutions is not maintained; it has been associated with users who prepare their own solutions and use of contaminated contact lens disinfection solution. Acanthamoeba is very difficult to eradicate because it is not susceptible to standard antiparasitic agents, and it produces a chronic keratitis that can be complicated by corneal perforation and loss of the eye. Corneal transplantation may be necessary in many cases to restore vision. In a study by Kaiserman and colleagues to assess the prognostic factors influencing visual prognosis and length of treatment after acanthamoeba keratitis, infection acquired by swimming or related to contact lenses, having neuritis and pseudodendrites, the absence of an epithelial defect, having been treated with chlorhexidine, and not having received steroids were factors that were associated with better final best spectacle-corrected visual acuity (BCVA); having an epithelial defect on presentation and having been treated with topical steroid were associated with worse BCVA.
Left Ventricular Assist Devices
The development plus use of mechanical circulatory assist devices has grown very rapidly in the past several decades, especially with the shortage of available donor hearts. Such devices have improved considerably the hemodynamic status and quality of life of patients with heart failure who are awaiting cardiac transplantation. Fig. 74.1 shows a left ventricular assist device (LVAD), a pneumatically driven pump located outside the heart that draws blood from an inflow cannula in the left ventricular apex and ejects the blood through an outlet into the ascending aorta. Within the pump are several sections of Dacron graft material and two trileaflet porcine valves in the inflow and outflow positions to ensure that blood flow is unidirectional. The pump is encased in titanium and implanted via an extended median sternotomy into the left rectus sheath. A percutaneous driveline connects to an exterior power pack for venting or for pneumatic actuation and exits the body after passing through a subcutaneous tunnel, in the left lower quadrant. The interior surface of the pump is textured to prevent the formation of thrombi and encourage the deposition of a biologic pseudointimal lining. Most LVADs are surgically implanted through a median sternotomy, with pump placement primarily in the intraabdominal or preperitoneal space. Ventricular assist device designs primarily fall into two major categories: pulsatile and continuous flow devices. Pulsatile devices are first-generation devices that were designed to mimic nature by providing pulsatile circulation; they have many moving parts, including a diaphragm to hold and eject blood, valves to maintain directionality, and an air vent and conduit for cooling of the working parts. These requirements have limited the durability and size of these devices. Because of the multiple moving parts, these devices begin to wear out within the first 2 years of use, and the large size necessitates placement in the preperitoneal space or intraperitoneal space. The second-generation continuous flow devices are smaller and have fewer moving parts. These devices primarily consist of inflow and outflow pumps and a rotor, with a smaller conduit to the power source that does not include an air vent. Since January 2010, 98% of LVADs implanted are of continuous flow design. Once the device is implanted, in most cases it cannot be removed without performing concurrent cardiac transplantation.

Infection of an LVAD can involve any portion of the LVAD, including the surgical site, driveline, pocket, and pump. Infections occur most commonly within the first 3 months of device implantation, with the risk for severe infection and sepsis peaking at 1 month. The cumulative risk for device infection increases the longer the device remains in place; the incidence of infection significantly decreases after 3 months. Based on data from the Interagency Registry for Mechanical Circulatory Support (INTERMACS), overall infection rates were 17.5 infections per 100 patient-months during the first 12 months after implantation. The rates of infection have been shown to vary among generations of device, with 28.9 infections per 100 months in the pulsatile devices ( n = 406) and 11.8 infections per 100 months in the continuous flow devices ( n = 548) ( P < .0001). It was also found that infection accounted for 16% of deaths associated with LVAD implantation.
The approach taken by many centers for infection of an LVAD includes both preventive and interventional steps that are instituted before, during, and after implantation. Preventive strategies are focused on prevention of infections related primarily to the driveline and device pocket through the use of clean implantation techniques and limited traffic in the operating room in which the procedure is being performed. Antibiotic prophylaxis usually is given for 48 hours near the time of implantation of the LVAD and may include intravenous trimethoprim-sulfamethoxazole, rifampin, and fluconazole, along with the application of mupirocin ointment to the nares. Additional preventive measures include soaking the surfaces of the LVAD in vancomycin and gentamicin for 30 minutes before placing it, irrigating the device pocket with povidone-iodine (Betadine), and meticulously placing the subcutaneous tunnel in the appropriate position. Postoperative care with sterile and semisterile dressing changes around the driveline is emphasized.
Despite the preventive measures taken, infection, thromboembolism, and hemorrhage at the time of implantation remain the most common complications associated with the use of mechanical circulatory support devices. Of these complications, infection has the most significant impact on morbidity and mortality. Predisposing factors for development of device-related infections in these patients include a variety of host, device, and operative characteristics. Device-related risk factors are correlated with increased size and surface area of the device, turbulence of flow, contact of blood with the prosthetic surfaces, device-related pockets and cavities, transcutaneous drivelines, and power cables. Operative and environmental risk factors include extent of surgery necessary for implantation hemorrhage, reoperation, frequent occurrence of multiple organ dysfunction or failure, duration of mechanical circulatory support, duration of hospitalization and intensive care unit stay, use of indwelling lines and catheters, parenteral nutrition, operative technique, and time. The incidence of infection in patients on LVAD support for longer than 60 days is reported to be two times higher than that in patients with an LVAD in place for less than 30 days; initial episodes of infection after 90 days are rare. With regard to patient-related risk factors, the severity of heart failure symptoms and presence of poor health and comorbid conditions, including diabetes, hyperglycemia, obesity, alcohol use, and renal disease, has been associated with increased risk for infection. In addition, some evidence indicates that implantation of an LVAD itself may lead to defects in cellular immunity secondary to an aberrant state of T-cell activation that predisposes LVAD recipients to the development of candidal and other systemic infections. The incidence of infection after implantation of an LVAD is reported to range between 13% and 80%, with most studies documenting an infection rate of 30% to 50%. Most series report that the device pocket and drivelines are the device-related sites that account for most of the infections. Placement of the LVAD in the abdominal cavity instead of the preperitoneal area has been shown to decrease the chance for development of infection.
Driveline infections are thought to result from a lack of integration of tissue around the driveline that allows the driveline to move and irritate surrounding tissues, which can result in an infection in the exit site and, in some cases, bacteremia. Such infections generally are defined by pain, erythema, warmth, drainage, or purulent discharge at the driveline exit site in the presence of a positive culture. Bacteremia developing after implantation of a pump and infections of the pump itself are additional reported complications. In a study of 205 patients who underwent placement of LVADs at the Cleveland Clinic, positive blood cultures were noted in 52%, infections of the driveline site occurred in 22%, and infections of the pump pocket developed in 9.2%. Sun and colleagues reported their experience with 95 patients who underwent insertion of an LVAD. Twenty-six (27%) of these patients experienced device-related infections involving the driveline, device pocket, or blood-contacting surfaces; 15 (57.7%) of the 26 had infections of the driveline site. Persistent bacteremia and progression of infection of the device pocket and driveline site can lead to the serious infection of LVAD endocarditis, which mimics prosthetic valve endocarditis, is very difficult to treat, and is associated with a high mortality rate. LVAD endocarditis is defined as positive cultures of the LVAD surface in conjunction with clinical signs and symptoms of infection during LVAD support. Manifestations of this condition are varied and range from persistent fever and bacteremia or fungemia to cerebral thromboembolism, LVAD inlet obstruction with hemorrhage, or LVAD outflow graft rupture. In a report by Weyland and associates of 27 patients who underwent insertion of LVADs, infections of the driveline developed in 8 (30%) of the 27 recipients, and LVAD endocarditis, defined as positive cultures from the pump chamber, was found in 12 (44%) of the patients; 8 (67%) of the 12 died of endocarditis.
Despite a high potential for the development of serious morbidity after placement of an LVAD, studies have shown that the presence of an infection after insertion of an LVAD does not seem to have very much influence on eventual cardiac transplantation and posttransplant outcome. A study by Argenziano found that infection subsequently developed in 29 (48%) of 60 patients who underwent insertion of an LVAD. The most frequent sites of infection were the blood (27%), LVAD driveline (13%), LVAD surface (13%), and central venous catheter (10%); these sites thus represented 63% of all infections. The overall mortality rate, the rate of successful cardiac transplantation, and the rate of infection after transplantation were not influenced by the presence of infection during LVAD support. In another study, Sinha and colleagues reviewed their experience with 86 patients who received LVADs; device-related infections developed in six patients, five (83%) of whom had infections of the pocket. This study also found that the presence of infection during LVAD support did not influence successful cardiac transplantation or patient survival after transplantation. Over a 6-year period, Schulman and colleagues reviewed their experience with 149 patients who received LVADs as a bridge to heart transplantation and sought to determine the impact of device-related infections on posttransplant survival and posttransplant infection. Of the 149 patients, 110 were successfully bridged to cardiac transplantation. Pretransplant device-related infections (e.g., pocket infections, wound infections, and postimplant bacteremia) had no negative effect on bridging to transplantation. However, patients with pretransplant sepsis were less often successfully bridged to cardiac transplantation. The presence of a pretransplant device-related infection was found to have no effect on posttransplant 1-year survival rates, although it was associated with an increased rate of posttransplant infection, especially if the patient had a driveline infection during LVAD support. Specifically having a driveline infection during LVAD support predisposed for having an infection in the former LVAD pocket or driveline site after cardiac transplantation, but did not predispose to having sternal wound infections, bacteremia, or sepsis in the posttransplant period. Diagnosing an infection early, determining the location and the extent of the infection, and assessing therapeutic efficacy can be a challenge in patients with LVADs. Anatomic imaging modalities such as CT and MRI may have limited value because of the presence of metallic CT artifacts and contraindications to using MRI. New imaging modalities, such as radiolabeled leukocyte single-photon emission computed tomography (SPECT/CT), are being studied to assess the accuracy of these modalities in revealing both anatomic location and extent of infection in patients with an LVAD. Preliminary results of leukocyte SPECT/CT have shown it to be very accurate in diagnosing an LVAD-related infection.
Microbiology of Left Ventricular Assist Device Infections
Numerous organisms have been isolated from LVAD infections. In most series, S. aureus (including methicillin-resistant strains), S. epidermidis and other coagulase-negative staphylococci, and Enterococcus spp. are the organisms most commonly isolated from these infections, usually within the first 4 weeks after implantation. Other organisms such as Enterobacter spp., P. aeruginosa, Serratia marcescens, Klebsiella spp. , Stenotrophomonas maltophilia, Citrobacter koseri, other gram-negative organisms, and polymicrobial organisms were seen more commonly later in the clinical course. *
* References .
Fungal infections (e.g., Candida albicans, Candida parapsilosis, Aspergillus spp., and Mucor spp.) have been reported or observed as well and were responsible for approximately 16% of the infections. However, colonization with fungi occurs in 35% to 39% of patients with LVADs. Investigators suggested that treatment with broad-spectrum antibiotics (common in these patients) rendered them more susceptible to the development of fungal infection. When a fungal LVAD infection is suspected, signs of thrush, cutaneous rashes, esophagitis, retinal changes, peripheral embolization, and unexplained decrease in LVAD output should be investigated. The presence of yeast on Gram stain of the fluid surrounding the device and ultrasound showing increasing amounts of fluid around the device can be used to help confirm the diagnosis.Management of these infections, especially LVAD endocarditis, presents a major challenge, given that the device usually cannot be removed unless simultaneous heart transplantation is performed; moreover if transplantation is accomplished, multiple issues regarding the immunosuppressed condition of the patient are raised. In most cases, management involves a prolonged course (i.e., usually 4 to 6 weeks) of aggressive antimicrobial or antifungal therapy (or both) appropriate for the organism or organisms isolated, with meticulous attention given to skin care around the driveline exit site; debridement, drainage, surgical revision, and irrigation with povidone-iodine of infected wound sites; or, in certain instances, replacement of the LVAD unit or cardiac transplantation before completing antibiotic therapy if a donor heart becomes available and all blood culture results are negative.
Extracorporeal Membrane Oxygenation Circuits
Extracorporeal membrane oxygenation (ECMO) is a life support device used most commonly for treating severe cardiac and pulmonary failure in neonates and children. Patients undergoing repair for congenital cardiac lesions may require ECMO support (>1%). In addition a growing number of children are receiving ECMO support for acute decompensation from myocarditis; cardiomyopathy; post-cardiac arrest; acute respiratory distress syndrome; severe bacterial, viral, or aspiration pneumonia; and sepsis with hypotension thought to be due to cardiac output failure. Specific indications for neonates include meconium aspiration syndrome, congenital diaphragmatic hernia, persistent pulmonary hypertension, and others. Although the overall survival rate of children managed by ECMO has improved in recent years, it still is approximately 50% to 80%, and is lower in adults. Multiple cardiac and noncardiac factors are associated with increased morbidity and mortality rates. Patients with a single ventricle or residual cardiac defect after surgery have a less favorable ECMO outcome. Renal dysfunction, especially requiring hemodialysis, multiple organ system failure, initiation of ECMO in the operating room, blood product transfusion, mediastinal bleeding, ECMO circuit problems, and duration of ECMO for longer than 10 days also were predictors of increased risk for mortality. The infection rate for patients on ECMO ranges from 6% to more than 40%, with a relatively recent report of 10.2% in about 40,000 patients in the Extracorporeal Life Support Organization registry. Recent reports suggested a higher infection rate in older patients with culture-proven infections, at 6.1% to 10.1% in neonatal patients, 14% to 23% in pediatric patients, and 20.5% to 30.6% in adults. The rate of development of sepsis in neonates while on ECMO in one study was 4.2% and was associated with higher complications and mortality. Multiple risk factors for acquiring infection while on ECMO were identified, including indwelling vascular or urinary catheters, duration of ECMO bypass (>10 days), procedures before or during ECMO, ECMO complications, and changes in the humoral and cellular immunity. Patients with infections usually will need longer bypass support and will have a delay in post-ECMO lung recovery and increased mortality. In one study, mediastinitis occurred in 11% of adults, with cardiac surgery noted to be a risk factor for this infection.
Earlier reports suggested that gram-positive cocci (e.g., S. aureus and coagulase-negative staphylococci) are the most common etiologic agents and are still frequent pathogens. However, gram-negative bacteria (both lactose fermenting and non–lactose fermenting ) and fungi are becoming more commonly reported. Candida spp. were found to be frequently isolated pathogens in children and adults. The distribution of these pathogens is similar to their distribution in other patients with infections treated in the intensive care unit. The signs and symptoms of infection often are subtle and nonspecific. Fever develops in only half of these patients. The value of tachycardia and elevated cardiac output also is limited because these patients frequently are tachycardic, with increased cardiac output caused by a systemic inflammatory response (e.g., complement; cytokines such as TNF, interleukin-1 [IL-1], and IL-6; and acute phase reactants such as C-reactive protein and procalcitonin), which is characteristic of cardiopulmonary bypass procedures. For the same reason, leukocytosis (unless developing acutely) and an elevated erythrocyte sedimentation rate (ESR) should be interpreted with caution. In addition, physical examination and radiologic tests may be difficult to interpret. Consequently a high index of suspicion with careful evaluation of potential infection source (e.g., surgical wound and insertion site of catheter) and surveillance of blood and urine cultures is needed to identify these infections.
Most patients undergoing ECMO are treated with multiple broad-spectrum antibiotics. This practice probably is one of the reasons for the increased incidence of multidrug-resistant, gram-positive (e.g., Enterococcus ) and gram-negative bacteria, as well as fungal (e.g., Candida ) infections. In addition, many of these patients will be colonized with these same bacteria. Therefore if a deep bronchial suction or urine culture (performed routinely) becomes positive but without supporting evidence of infection (e.g., increased white blood cell [WBC] count, fever), serious effort to distinguish between colonization and infection should be made before initiating an unnecessary change in antimicrobial therapy. If a decision to treat is made, appropriate coverage for both gram-positive and gram-negative organisms should be chosen. If blood, urine, and bronchial cultures remain negative and the patient’s condition is not improving or the culture becomes positive for a fungus, antifungal therapy should be added.
Most ECMO centers administer antibiotic prophylaxis; however, the optimal spectrum and duration of this practice are unknown. Some studies suggested that use of prophylaxis (for 24 hours before the ECMO is started) was effective in reducing infections ; others found no correlation between antibiotic prophylaxis and reduction in infection rate. Of interest, despite advances in critical care for ECMO patients (including antibiotic prophylaxis), no significant decrease has occurred in infection rate during the past two decades. The lack of specific guidelines and evidence from randomized trials evaluating different durations and spectrums of antibiotic contribute to the confusion and controversy in this area. If prophylaxis is considered, antifungal prophylaxis (e.g., fluconazole) should be added because it was shown to decrease the incidence of fungal infections, which are increasing.
Permanent Cardiac Pacemaker and Implantable Cardioverter-Defibrillator Infections
Permanent Cardiac Pacemaker Infections
Cardiac pacemakers have been widely used since the 1980s and the American Heart Association (AHA) has recently expanded the indications for their use. Two types of permanent pacemaker generators are in use; those with transvenous electrodes and those with epicardial electrodes. Both types are implanted in either the chest or the abdominal wall; pacemakers with epicardial electrodes are the ones more commonly used in the pediatric population. Studies are currently being conducted on the next generation of pacemakers, which are miniaturized, fully self-contained leadless devices that are nonsurgically implanted in the right ventricle with the use of a catheter.
Infection is one of the most common medical complications of implantation of permanent pacemakers, with reported rates ranging from 0.2% to 5.8% and a high mortality rate. An estimated 25% of cardiac pacemaker–associated infections develop within 1 to 2 months of placement of the device, but delays of up to 12 months may occur before diagnosis is established. Several studies suggested that the relative rate of infection associated with permanent pacemakers has increased despite improved surgical techniques and better design of the devices themselves, but a recent study demonstrated a fairly constant rate of infection over the course of 20 years. Studies have shown that infections of permanent cardiac pacemakers seem to occur more commonly in patients with both local and systemic underlying medical problems, especially diabetes mellitus or an underlying renal insufficiency, or in those who are undergoing treatment with corticosteroids, anticoagulants, or other types of immunosuppressive therapy. Other well-characterized independent risk factors include surgery related to any part of the pacemaker (especially replacement of a battery or upgrade of the pacemaker), the presence of a hematoma after implantation, temporary transvenous pacing, and epicardial leads. Multiple (two or more) pacemaker insertions and physician inexperience with insertion also represent significant risks for development of infection. The presence of a central venous line also increases the risk for systemic infection. Multivariable analysis of the largest reported pediatric pacemaker infections identified only presence of Down syndrome and manipulations of the pacing system during revisions as risk factors.
Infections of permanent cardiac pacemakers are subdivided into groups depending on the specific site of involvement and whether they are early infections (<1 to 2 months after implantation) or late infections (>2 months after implantation). The groups are (1) local inflammation, infection, and the formation of an abscess in the generator pocket or subcutaneous portion of the lead, or in both sites; (2) secondary infection involving either the generator or the electrodes (including pacemaker endocarditis) ; (3) fever plus associated bacteremia, with or without concomitant infective endocarditis, in a patient without any apparent focus of infection ; and (4) mediastinitis, pericarditis, bronchopleural cutaneous fistulas, and mixed infections.
The generator pocket and the subcutaneous portions of the leads (i.e., transvenous and epicardial) are the most common sites of infection. Infections of the pocket often are difficult to diagnose and may develop at any time after implantation; however, most tend to develop early and frequently are the result of contamination by skin flora at the time of the procedure. Late infection usually occurs as a consequence of erosion of the device through the subcutaneous tissue and skin. The infection may remain localized to the pocket or may spread to the adjacent electrodes and lead to the development of bacteremia. Infection of transvenous pacemaker leads may occur in as many as 17% of patients who receive permanent pacemakers. These infections often are not apparent initially but have been associated with significant morbidity and mortality. Infection generally develops along the subcutaneous portion of the leads and, if unrecognized, may progress centrally and result in sustained bacteremia, endocarditis, or both. Infections of the leads tend to occur later than infections of the pocket, with the median time of occurrence being 7 to 8 months after implantation of the pacemaker. Endocarditis as a complication of unrecognized infected transvenous leads can develop years (2 to 3 years) after placement of the pacemaker. Infections of permanent epicardial leads generally occur as a complication of infections of the generator pocket, skin erosion, or direct contamination at the time of placement. However, in contrast to infections of the transvenous leads, infections of the epicardial leads usually result in only local symptoms; rarely they may lead to more severe disseminated disease such as pericarditis, mediastinitis, bronchopleural cutaneous fistulas, bacteremia, or sepsis.
Microbiology
Staphylococci ( S. aureus and coagulase-negative staphylococci) are the most common causes of pacemaker-related infections, accounting for more than 85% of such infections; however, a wide variety of organisms have been isolated. An increasing number of the isolated staphylococci are methicillin resistant. Other gram-positive organisms such as viridans streptococci, other streptococci, enterococci, Listeria, and Corynebacteria have been isolated as well, but each causes fewer than 1% of cases. Gram-negative organisms such as E. coli, Proteus, Enterobacter cloacae, P. aeruginosa, Klebsiella, S. marcescens , and Sphingomonas paucimobilis are isolated from 5% to 20% of device-related infections. In addition, Candida, Aspergillus, Pseudallescheria , Trichosporon spp., and other fungi have been isolated rarely, as well as Mycobacterium spp.; however, infections with these organisms generally are associated with poor clinical outcomes. *
* References .
Clinical and Laboratory Findings
Diagnosing a pacemaker-associated infection can be difficult because of its nonspecific symptomatology, and months may elapse after the onset of symptoms before the diagnosis is established. Symptoms may be confined to a local area or may be more widespread, with the development of bacteremia or other systemic effects. Rarely the only sign of infection is persistent pain at the pocket site. Fever (84–100%) and chills (75–84%) are considered the most common systemic symptoms and are indicators of local infection, especially if they occur after the second postoperative day in association with other signs; however, they may be the only clinical manifestation in more than a third of patients. Early infections are more likely to be accompanied by both local and systemic clinical findings and are manifested more commonly as infections of the generator pocket, bacteremia, or septicemia ; late infections, on the other hand, typically cause vague symptoms that evolve over the course of time and usually are lead-related infections and endocarditis.
Infections of the generator pocket typically cause local swelling (21%), erythema (34%), pain (32%), drainage (through an incompletely healed incision or fistulous tract) over or adjacent to the generator pocket (25%), and warmth (11.5%). Sterile breakdown of the pacemaker pocket develops in an estimated 5% of patients with permanent pacemakers, and skin or soft tissue erosion over the electrodes occurs in an additional 2% to 4%. Because these complications are associated with a high risk for the subsequent development of infections, the presence of any erosion is considered a potential indicator of device-related infection, especially if the erosion occurs more than 24 months after placement of the pacemaker, at which time the infection rate may be as high as 80%. Pacemaker-related endocarditis is a relatively rare complication that occurs in 0.05% to 0.5% of cases after implantation of a pacemaker. Such endocarditis is associated with a mortality rate as high as 34% in some series. It tends to be a late complication; only 27% to 36% of patients are seen within 6 to 12 weeks after the last procedure at the pacemaker implant site, with symptoms developing in most patients a mean of 25 months after the last procedure at the implant site. The diagnosis of pacemaker-related endocarditis is difficult to establish, and usually a delay occurs in making the diagnosis, with a mean interval of 5 to 8 months after the onset of symptoms. Pulmonary symptoms are found in 20% to 45% of patients with pacemaker-related infections and may consist of bronchitis, lung abscess, pneumonia, or pulmonary embolism; these symptoms are seen more commonly in late-onset infection and in patients with intravenous lead–related infection.
A variety of laboratory and imaging studies may be performed to aid in establishing the diagnosis of a pacemaker-related infection. A recent update from the AHA suggested two sets of cultures from blood, generator pocket site, and lead tips when infection is suspected. A prospective study that looked at patients with pocket infections of cardiovascular implantable electronic devices found that sonication of generators and leads that were surgically removed was more sensitive than swab cultures or blood cultures alone in detecting bacteria in these types of infections. An elevated ESR is found in 82% to 97% of patients, and peripheral leukocytosis is seen in 50% to 66%. The presence of a collection of fluid around the device as seen on ultrasound is suggestive of device-related infection. Gallium scanning may be performed in an attempt to determine the nature of the fluid (i.e., inflammatory vs. infectious). Transesophageal echocardiography (TEE) can be helpful in identifying endocarditis (especially when blood culture results are negative). TEE has emerged as a major tool in diagnosis; studies have shown that this test was able to demonstrate vegetations and other lesions on the electrodes, ventricular endocardium, and tricuspid valve in 90% to 96% of patients with pacemaker-related infections. Only the combination of negative TEE and ECHO should be used to rule out endocarditis.
Management and Treatment of Infection
Appropriate therapy for pacemaker-related infections is tailored to the specific clinical situation and depends on several factors: (1) whether the infection is limited to the generator pocket or subcutaneous electrodes, without bacteremia; (2) whether bacteremic pacemaker-related endocarditis is present with or without involvement of the subcutaneous electrodes; and (3) what organism is involved.
If the infection is limited to the generator pocket or subcutaneous electrodes, optimal treatment consists of both medical and surgical intervention in which all of the parts of the infected device are removed. Studies have shown that failure to remove completely all portions of the infected device results not only in failure to cure the infection but also in higher mortality rates. In addition, infection relapse is higher. The patient is given parenteral antimicrobial therapy, and an exchange of the pacing system with total removal of the infected device and simultaneous insertion of a new pacemaker at a different site is performed surgically. The infected subcutaneous pocket is drained, debrided, and packed open, and local wound care is instituted. Initial antibiotic therapy should provide coverage for both staphylococci and gram-negative bacteria; therapy is tailored once the organism is identified and its antibiotic susceptibility is known. Initial regimens include vancomycin plus an aminoglycoside. Oxacillin or nafcillin plus an aminoglycoside may be used if MRSA is rare in the community. In certain instances, a new pacemaker cannot be placed at the time of removal of the infected device; in such situations, a period of temporary transvenous pacing is instituted until a new pacemaker can be implanted. For local pocket infections, antimicrobial therapy targeted at the causative organism should be administered for 10 to 14 days.
The consensus approach to the management of pacemaker-related endocarditis and bacteremia involves removal of both the generator unit and the electrodes in conjunction with prolonged administration of parenteral antibiotic therapy designed to treat endocarditis (usual duration, 4 weeks). In cases of MRSA, duration of treatment should be extended to 6 weeks. A longer duration may be considered in patients with other sites of infection (e.g., osteomyelitis or septic thrombophlebitis). Vancomycin should be the initial antimicrobial treatment of choice before the etiologic agent is identified because the majority of infections are due to staphylococci species. More specific antibiotic regimens can be used when the pathogen is identified and in vitro susceptibility is available. Mortality rates may be as high as 50% for patients in whom total removal of the pacemaker unit is not performed. Percutaneous removal of electrodes that have lead-associated vegetations smaller than 20 mm is acceptable, but extraction of the electrodes with vegetations larger than 20 mm may be difficult and risky to perform, and surgical removal by cardiotomy may be necessary. In patients who require a pacemaker, temporary transvenous pacing may be implemented until a new permanent pacemaker can be placed. Sterility of blood cultures should be ensured before a new pacemaker generator and electrode are placed.
Infection of Implantable Cardioverter-Defibrillators
The first implantable cardioverter-defibrillator (ICD) was placed in 1980, and, during the past several decades, the device has become a successful therapeutic modality for treatment of patients (both adults and children) with life-threatening ventricular arrhythmias. Older devices required surgical placement of a pulse generator, extrapericardial or epicardial defibrillation patches, and a transvenous rate-sensing electrode. More recent systems use transvenous approaches for placement of leads and a subcutaneous or submuscular pulse generator or newer methods of complete subcutaneous ICDs, including subcutaneous leads.
Infection is the most serious complication of placement of an ICD. Infection rate ranges associated with placement of ICDs were previously from 1% to 7%. However, infection rates have decreased substantially with the advent of transvenous non–thoracotomy-placed systems, with rates ranging from 0.2% to 1.8% and 3.1 per 1000 patient years. Subcutaneous ICDs appear to have lower rates of infection, particularly endocarditis. Results from some studies still suggest that ICDs are associated with a greater risk of infection than are permanent pacemakers. Cost associated with hospital admission, length of stay, and mortality are substantially increased in patients with infection in contrast to those without it. Several risk factors that have been identified as increasing the likelihood for development of infection include steroid use, diabetes, heart failure, renal failure, malignancy, chronic obstructive pulmonary disease, skin disorders, anticoagulant drug use, preprocedural fever, and previous device infection. Previous generator replacement, abdominal implantation, postoperative hematoma, procedural duration, and surgeon experience also were reported as contributing to increased risk for infection. Abnormal renal function is the most consistently identified risk factor for mortality. Mortality is high, ranging from 24.5% to 29%, and is higher for patients with infective endocarditis or bloodstream infection compared to generator pocket infection only.
Most ICD-related infections are clinically apparent within 3 to 6 months after placement, with infections of the generator pocket, subcutaneous patch wound site, epicardial patches, or bacteremia and endocarditis being the most common manifestations. Some infections can be indolent, and only a high index of suspicion will initiate an investigation. An increased WBC count and ESR, anemia, and microscopic hematuria are the most common laboratory findings. As with pacemaker infections, TEE is an effective test to exclude endocarditis because transthoracic echocardiography is helpful in only half of the cases. Infection of the generator pocket or subcutaneous patch wound site usually causes local findings of pain, erythema, and collection and drainage of fluid. Occasionally these patients also may be bacteremic or hypotensive. In contrast, infection of the epicardial patches generally results in more systemic symptoms, bacteremia, or pericarditis. At least two blood cultures should be obtained to rule out bacteremia. Cultures of the generator pocket and lead tips after removal of the device are essential for identifying the pathogen. Sonication of the extracted device increases the yield of positive cultures.
Microbiology
Gram-positive bacteria, primarily coagulase-negative streptococci and S. aureus are the major pathogens seen with ICD-related infections and account for 68% to 93% of cases. *
* References .
However, a broad spectrum of other gram-positive, gram-negative, and fungal organisms, including Enterococcus spp., P. aeruginosa, Corynebacterium, streptococci, E. coli, Klebsiella , Bacteroides fragilis, Propionibacterium acnes, atypical mycobacteria, and Candida spp., have been isolated from ICD-related infections. Fungal infection is uncommon, reported in fewer than 2% of patients. Reported rates of polymicrobial infection range from 2% to 24.5%, and many patients with clinical infection are found to have negative cultures (12–49%).
Management
The same approach previously described for pacemaker infection should be applied to ICD infection. To eliminate ICD-related infections effectively, optimal management involves a combination of medical and surgical interventions, especially if the patient is bacteremic or has systemic findings or if the causative organism is S. aureus . Several studies have suggested that conservative management (i.e., antibiotic therapy without removal of the hardware) may be sufficient, but others have identified medical therapy as a risk factor for death. A medical management approach should be tried only in cases with superficial or incisional infection and in rare occasions when only the generator pocket is infected or in patients whose condition prevents removal of the entire device. In these cases, the treatment should consist of administration of parenteral antibiotics, wound care, and removal of only the generator portion of the device, with implantation of another generator at a different site. The optimal management of any other ICD-related infection is parenteral antibiotics and complete removal of the entire ICD system, followed by reimplantation (after the infection is eradicated) at another site. Although there are no clinical trial data to define the optimal duration of antimicrobial therapy, at least 2 weeks should be considered after hardware removal, and, if blood cultures continue to be positive 24 hours after removal of the ICD, a longer period (e.g., 4 weeks) should be considered. For infections limited to the pocket site, 7 to 10 days of appropriate antibiotics may be sufficient, with an oral agent being a reasonable option if there is only superficial or incisional involvement.
Prosthetic Joint and Orthopedic Implant Infections
The implantation of prosthetic joints along with the use of other implantable orthopedic devices (e.g., pins, screws, plates, rods, external fixators, or Ilizarov apparatus) has improved quality of life greatly and restored function to patients suffering from debilitating bone and joint disease, tumor, or injury. Based on conservative estimates, millions of people worldwide have some form of prosthetic joint or other implantable orthopedic device. Of the possible complications associated with implantation, infection is the second most common cause of prosthetic joint failure. It occurs in 0.6% to 2.4% of total joint replacement and results in postoperative prosthesis failure, chronic pain, immobility, and, in some cases, loss of the affected limb or, in the worst-case scenario, loss of life. The health care cost in the United States for treating a single infection of a prosthetic joint is estimated to be more than $280 million per year nationwide. The number of prosthetic joint infections is increasing.
Prosthetic joints and implantable orthopedic devices may become infected by two major mechanisms: (1) the prosthetic device may be contaminated by microorganisms at the time of implantation, either as a result of airborne contamination in the operating room or by direct inoculation at the time of surgery; or (2) the prosthetic device may become infected as a result of hematogenous seeding from bacteremia or by direct contiguous spread from an infection adjacent to the prosthesis. Of infections of prosthetic joints, 20% to 40% arise by hematogenous seeding, with the remainder occurring as a result of airborne or direct inoculation. Infections may remain asymptomatic for years before symptoms become apparent, and usually a long delay occurs between onset of the infection and the appearance of symptoms and confirmation of the diagnosis. The overall rate of infection of prosthetic joints has been shown to be highest in the first 6 months postoperatively, with a steady decline occurring after this time. The incidence rate for infection of total hip and total knee arthroplasties during the first 2 years postoperatively is reported to be 5.9 infections per 1000 joint-years; in contrast, during postoperative years 2 to 10, it is 2.3 infections per 1000 joint-years. The risk for acquiring infection and the incidence of infection depend on the anatomic location of the implanted orthopedic device or prosthetic joint, with the hips having the highest risk, followed in descending order by the knees, elbows, shoulders, wrists, and ankles. For implantable orthopedic devices, rates of infection range from 2% to 30%.
Risk Factors
Numerous factors have been identified as increasing a patient’s risk for developing infection of a prosthetic joint or orthopedic implant, including rheumatoid arthritis, diabetes mellitus, obesity, poor nutritional status, use of steroids, immunocompromised status, psoriasis, hemophilia, sickle-cell hemoglobinopathy, solid-organ transplant, dialysis-dependent renal failure, joint dislocation, and extremes of age. In addition, procedure-related factors such as previous surgery at the site of the prosthesis or implant, extended operation time (>2.5 hours), postoperative bleeding, and hematoma also increase the risk for acquiring an infection. The relative risk for the development of prosthesis-related infections in patients with poor healing and wound complications increases from 13- to 20-fold after total knee replacement and from 22- to 52-fold after total hip replacement. In addition, bacteremia from any source increases the risk for prosthesis infection. For example, prosthesis-related infection developed in 34% of patients with S. aureus bacteremia who were followed prospectively.
The implanted metal prosthetic device and the PMMA cement that binds the prosthetic device to adjacent bone also predispose the joint space and bone to infectious processes, given that both are foreign bodies. In vitro studies have shown that the unpolymerized form of PMMA cement predisposes to infection by inhibiting phagocytic, lymphocytic, and complement function; the risk for acquiring infection seems to be enhanced further once the cement has polymerized in the body. Cementless prostheses have been designed in an attempt to overcome the problem of infection associated with the PMMA cement. For certain orthopedic implants, the integrity of the skin is compromised chronically, thus providing ready access to organisms from the external environment.
Microbiology
Although any microorganism can cause prosthetic joint infections, more than half of the infections are caused by S. aureus or coagulase-negative staphylococci. Other gram-positive bacteria (e.g., β-hemolytic streptococci, viridans streptococci, and enterococci) cause 20% to 25% of infections. Antibiotic resistance in these organisms is increasing, and multiple strains of staphylococci may be present in a single prosthetic joint infection. Less commonly, aerobic gram-negative bacilli, including E. coli, Proteus mirabilis, Klebsiella spp., Salmonella spp., S. marcescens , other Enterobacteriaceae, and P. aeruginosa , may cause infection. In addition, 4% to 10% of infections are caused by anaerobic organisms such as peptostreptococci and Bacteroides spp.; polymicrobial infections occur in approximately 10% of cases, with S. aureus and anaerobes the most frequently found. Infections with fungi, particularly Candida, Aspergillus, and Penicillium spp., or with mycobacteria (i.e., Mycobacterium tuberculosis, Mycobacterium avium complex [MAC], and other rapidly growing mycobacteria) also have been described. Rarely a wide spectrum of other organisms, including Corynebacterium, Propionibacterium, Bacillus spp., and Mycoplasma hominis, have been reported to cause infection. Zoonotic bacteria such as Brucella spp., Yersinia spp., and Pasteurella spp. rarely can cause infection and should be considered in the correct epidemiologic setting. Approximately 10% of infections are culture negative.
Certain clinical situations may predispose a patient to particular organisms as the cause of infection. Pyogenic skin infections commonly result in staphylococcal and streptococcal infections of prosthetic joints, whereas infections of the teeth and gums are frequent causes of viridans streptococcal and anaerobic infections in prostheses. Genitourinary and gastrointestinal tract procedures or infections frequently are associated with enterococcal and gram-negative bacillary infections of prostheses. The increased use of TNF-α blockers in patients with joint prosthesis predisposes them to infections with unusual pathogens (e.g., fungi or mycobacteria).
Clinical Manifestations
The clinical findings and severity of symptoms seen with infections of prosthetic joints are highly variable and determined primarily by three factors: (1) the route of infection—the hematogenous route versus direct inoculation; (2) the virulence of the infecting pathogen— S. aureus and, to a lesser extent, β-hemolytic streptococci and gram-negative bacilli seem to be particularly virulent pathogens capable of producing a fulminant clinical picture, whereas infection with organisms such as coagulase-negative streptococci is associated with a more chronic, indolent course; and (3) the nature of the tissue in which the microorganism proliferates—hematomas, seromas, ischemic wounds, and the tissues of diabetic patients and those receiving steroids all enhance the ability of the bacteria to proliferate and spread, thereby promoting the development of a more deep-seated fulminant infection.
The most common initial symptom is joint pain, which occurs in 95% of cases. Such pain can range from an acute fulminant illness with erythema, severe joint pain, swelling (38%), high fever (43%), and systemic symptoms to a chronic, slowly increasing pain in the joint without typical signs of inflammation that may be associated with the formation of a cutaneous draining sinus (32%) but no systemic symptoms. The presence of constant joint pain is more indicative of infection than is the presence of pain occurring only with movement or weight-bearing, which is more common in aseptic and mechanical complications.
For implantable orthopedic devices, the most common initial symptom of infection is erythema, swelling, pain, or drainage from the area around and adjacent to the implant. Local symptoms also may be associated with fever, especially if the device is extensive or deep seated.
Diagnostic Studies
Laboratory screening tests commonly used to diagnose infection of a prosthetic joint or implanted orthopedic device such as peripheral WBC count, C-reactive protein (CRP), and ESR are neither specific nor sensitive (sensitivity, 60–96%; specificity, 55–92%). Of these tests, CRP seems to be the better test. These test results may be normal, especially in patients with chronic, slowly progressing infection. Several biomarkers (e.g., procalcitonin, TNF, and IL-6 ) have been suggested as diagnostic tests. Molecular techniques such as polymerase chain reaction (PCR), fluorescence in situ hybridization (FISH), and immunofluorescent microscopy were reported to have a sensitivity of 63% to 100% in detecting bacteria in prosthetic joint infections but were not studied widely.
The principal radiologic studies used for detection of an infected prosthetic joint include plain radiographs, arthrograms or sinograms, and radioisotope scans (indium and technetium diphosphate). Abnormalities that may be suggestive of infection and can be seen on plain radiographs include radiolucency at the bone-cement interface, motion and changes in position of the prosthetic components, evidence of osteomyelitis, cement fractures, and periosteal reaction. Intraarticular injection of dye (arthrogram or sinogram—in the presence of a sinus tract) may demonstrate abnormal communication between the joint space and the bone–cement interface. These radiographic abnormalities are present in approximately 50% of infected prostheses. Nuclear scans with indium-111–labeled WBC or technetium-labeled sulfur colloid ( 99m Tc) may be used to detect periprosthetic inflammation. Scanning techniques have a sensitivity of 80% to 86% and a specificity of 94% to 100%. Of note, increased uptake can be seen around uninfected prostheses within the first 6 to 12 months after implantation. Therefore positive findings after this time are abnormal and reflect inflammation (which could be due to a variety of causes) but not specifically infection. Of interest, 99m Tc-labeled ciprofloxacin (which probably binds to live bacteria at the infection site) was shown to be 94% sensitive and 83% specific for diagnosis of chronic infection. Use of this technique allowed 11 of 12 infections of prosthetic joints to be identified, whereas 99m Tc-WBC scintigraphy was positive in only seven patients. Combining antigranulocyte antibody ( 99m Tc sulesomab) and 99m Tc collodial rhenium sulfide (nanocolloids) was shown to be an acceptable alternative. Positron emission tomography with fludeoxyglucose F-18 (18 FP-FDG PET) was also evaluated as a diagnostic technique. CT and MRI are not used routinely in the evaluation of patients with suspected infection of a prosthetic implant because of the large amount of imaging artifact created by prosthetic devices.
Aspiration of joint fluid for culture and culture of tissue obtained intraoperatively are the optimal ways to establish a specific diagnosis of infection of a prosthetic joint. Unfortunately the yield is only 50% to 90%. To optimize the yield, antibiotics should be stopped 2 to 3 days before aspiration or operative cultures are taken. Although joint fluid findings indicative of an infectious process are a high leukocyte count (consisting mainly of polymorphonuclear leukocytes [PMNs]), a high protein content, and a low glucose concentration, these changes are not specific for bacterial infection and are present in only some patients. Using a cutoff value of 1700 WBC/µL with at least 65% PMN cells gave excellent results. The fluid obtained should be cultured for a variety of organisms; Gram stain is positive in a third of cases, and a causative pathogen can be identified in only two-thirds of patients. Intraoperative cultures should include, if possible, any purulent discharge, devitalized bone, and tissue from the bone–cement interface. Vortexing, bath sonication, or both of the explanted prosthesis may increase the yield. Histopathologic examination of this tissue usually reveals an infiltration of PMNs consistent with an acute inflammatory reaction but not specific for infection. More rapid, specific, and sensitive tests (e.g., FISH, PCR, immunofluorescent microscopy) are needed to differentiate between noninfectious inflammation and bacterial infection and identify the pathogen in patients already receiving antibiotic or infected with fastidious pathogens.
Treatment
Successful treatment of an infected prosthetic joint involves extensive surgical debridement of all devitalized bone and tissue, removal of the prosthesis and all associated cement, and prolonged parenteral antibiotic therapy. For optimal results, the debridement should be performed within 1 to 3 weeks. Microbiologic cure has been found to correlate well with the extent of debridement and the completeness of removal of all residual methylmethacrylate cement. Historically attempts at simple surgical debridement without removal of the prosthetic device in conjunction with parenteral antibiotic therapy had variable success, with relapse rates being as high as 88% by 2 to 4 years after therapy. High failure rate was reported in S. aureus infections, but in patients with a short duration of prosthetic joint infection caused by penicillin-susceptible streptococci, debridement and antibiotic therapy alone appear to be sufficient, with a low risk for relapse.
Two protocols have been used for the treatment of these infections. A two-stage surgical procedure with prolonged parenteral antibiotic therapy has been shown to be one of the most successful treatment regimens with the best functional results. The first stage involves complete removal of the prosthesis and cement followed by a 6-week regimen of antibiotic therapy empirically chosen to cover the most likely organism and then tailored once the organism has been identified and its antibiotic susceptibility is known. The second stage involves reimplantation of a new prosthetic device at the end of the antibiotic course. Success rates with this procedure range from 87% to 100%. An alternative method of therapy involves a one-stage exchange operation in which the infected prosthetic device and cement are removed, all devitalized tissue and bone are debrided, and a new prosthesis is reimplanted immediately, followed by 6 weeks of parenteral antibiotic therapy. Antibiotic-impregnated (i.e., tobramycin or gentamicin) methylmethacrylate cement is used in these situations, and success rates of more than 75% were reported. This procedure is appropriate only for patients with infections caused by less virulent microorganisms because of the high failure rates seen when a more virulent organism such as S. aureus or a gram-negative bacillus is the cause of the infection. The Ilizarov method allows simultaneous treatment of infection, bone and joint deformities, bone loss, and shortening of the limb. This device includes proximal and distal circular external rings with wires passing through the bone and soft tissue from one side of the limb to the other. Although this method is used more often for the treatment of bone nonunion or bone loss with or without infection, David and colleagues used this technique successfully to treat 12 patients who failed total knee arthroplasty because of infection. Wound infection and chronic osteomyelitis caused by infection of the wire tract occur infrequently and should be treated by debridement of the infected soft tissue and curettage of the infected bone.
The two-stage prosthetic removal-reimplantation procedure coupled with the incorporation of antibiotic-impregnated cement during reinsertion of the implant, in combination with a 6-week antibiotic regimen, is the mainstay of therapy for infections of prosthetic joints. Selection of antibiotics should take into account the recent increase in methicillin resistance among staphylococci for the following reasons: MRSA infections of the prosthetic joint result in a higher incidence of treatment failure than do infections with methicillin-susceptible S. aureus , and the former result in longer durations of hospitalization and a low survival rate free of treatment failure. Thus vancomycin (with or without rifampin) should be the initial drug of choice for gram-positive bacteria until the susceptibility of the infecting organism is known. In chronic and hematogenous infections (in which gram-negative bacteria are more common) carbapenems, aminoglycosides, or fluoroquinolones should be considered in addition to vancomycin until the microbiology results are available. Reports of treatment failures (even with vancomycin susceptibility within the treatment range [i.e., 2 to 4/µg per mL]) suggest that other antibiotics (e.g., trimethoprim-sulfamethoxazole or linezolid) should be considered. Several reports have shown that linezolid is a reasonable alternative that results in control of the infection.
For infected implanted orthopedic devices, the success of therapy is based on total removal of the device together with parenteral antibiotic therapy. In cases in which the foreign body cannot be removed, extended parenteral antibiotic therapy should be instituted and continued until the device can be removed. The duration of therapy varies with the severity of the infection and ranges from several weeks to several years.
Prevention
Like other surgical procedures, prevention efforts should be focused on reducing the ability of the pathogen to reach the surgical site. Patients should be screened for S. aureus colonization, and efforts should be made to decolonize those found to be carriers. The success of intranasal mupirocin or bathing with chlorhexidine in reducing the rate of surgical site infections suggests that such measures should be considered for patients receiving prosthetic joint or other implantable orthopedic devices.
The use of prophylactic antibiotics for dental procedures in patients with an orthopedic device is controversial. Although no evidence supports such prophylaxis, the American Academy of Orthopedic Surgery recommended it for all patients with joint implants. The practice of using antimicrobial-loaded bone cements for prophylaxis is also controversial. Retrospective studies found it to be effective, but prospective studies found no extra benefit compared to systemic antibiotic prophylaxis. Animal models looking at the use of new biodegradable materials to replace antibiotic impregnated poly(methylmethacrylate) bone cement have shown promise in reducing the number of follow-up surgeries, enhancing antimicrobial efficacy, and regenerating bone in an infected defect.
Cerebrospinal Fluid Shunts
Infection is a major cause of morbidity in children who undergo CSF shunting procedures. Such procedures are performed to divert CSF in symptomatic hydrocephalic patients and are used commonly in patients with anatomic abnormalities (e.g., meningomyelocele and Chiari malformations), in premature newborns with intraventricular hemorrhage or intracranial infections (e.g., congenital cytomegalovirus, congenital toxoplasmosis, and bacterial meningitis), and in patients with central nervous system (CNS) tumors or head trauma. Usually the proximal end of the shunt is placed in the frontal or fourth ventricle and the distal end is inserted into the peritoneal cavity (i.e., ventriculoperitoneal shunt). Other compartments such as the right atrium (i.e., ventriculoatrial shunt), the pleural cavity, or the gallbladder can be used to place the distal end. CSF shunts are prone to complications, with a 10-year failure rate greater than 50%. The most common complication is mechanical (such as obstruction or overdrainage), followed by shunt-related infection. The incidence of shunt-related infection varies considerably, from 0.3% to 26%, with more recent studies reporting a range of 8% to 12%. These infections increase morbidity rates and, in some cases, significantly affect patient outcomes. In addition, the increased morbidity caused additional expenses and resulted in higher costs to the health care system. Understanding some of the factors that contribute to the development of these infections has helped reduce their incidence.
Epidemiology
Almost two-thirds of shunt-related infections occur within 1 month after placement of the shunt, and 90% of infections are manifested within 6 months. The incidence of shunt-related infections is significantly higher in infants in the first 6 months of life than in older children or adults. The infection rate is even higher in newborns with intraventricular hemorrhage who undergo shunting in the first week of life and in premature infants. Reasons for the increased incidence of shunt-related infection in very young patients are multifactorial. Several mechanisms that have been suggested include delayed wound healing, higher skin density of bacteria that are more resistant to antibiotics and more adherent to the shunt, longer duration of hospitalization, surgical technique, and increased exposure to antibiotics just before the shunt placement.
No significant difference in infection rate is seen in patients with ventriculoperitoneal or ventriculoatrial shunts, but a lower infection rate was noted in those with lumboperitoneal or cholecystic shunts. An increased risk for development of infection was reported for shunts placed immediately after removal of a previously infected shunt, probably because of incomplete eradication of bacteria. Several other factors, including the underlying cause of the hydrocephalus, the surgeon’s experience, previous shunt infection, the duration of surgery, the number of people in the operating room, perioperative factors (e.g., prophylactic antibiotics, skin preparation, and shaveless operation), operative time, open surgery to insert the abdominal catheter versus direct puncture of the abdominal wall with a trocar, postoperative CSF leakage, and others, have been reported to be associated with an increased incidence of shunt-related infection. Although a trend toward more shunt-related infections has been reported with these factors, it has not been demonstrated consistently. Malignant lesions and trauma as etiologies may have lower rates of infection. A few studies suggest that tapping the shunt may result in an infection, with an incidence ranging from negligible to greater than 30% in premature infants with shunts tapped multiple times. Reinfection rate was estimated to be around 25%. Multiple factors were suggested as contributing to reinfection, including treatment duration of the first infection, age younger than 6 months, nonsurgical management, intraventricular hemorrhage (IVH) of prematurity, intracranial cysts, or aqueductal stenosis. The median time to reinfection (i.e., second episode) ranged from 65 to 90 days. Almost 60% of children with second infection developed a third reinfection, and 47% of them developed a fourth infection. The median time to the second infection was 65 days, for the third infection 477 days, and for the fourth infection 2137 days.
Etiology
Staphylococcal species are the most common cause of shunt-related infection, with coagulase-negative staphylococci (e.g., Staphylococcus epidermidis, S. capitis, S. hominis, and S. lugdunensis ) being isolated in 25% to 70% of cases ( Table 74.1 ). S. aureus, the second most common gram-positive bacterium, is responsible for 10% to 40% of cases. Streptococci (e.g., viridans, group B or C, S. pyogenes, S. pneumoniae, enterococci) are identified less commonly (3–7%). Other gram-positive bacteria such as Propionibacterium and Corynebacterium (diphtheroids) are isolated as well. The seemingly increased incidence of shunt-related infections caused by these two groups of bacteria probably is the result of poor culture technique (e.g., failure to use anaerobic culture media, less than 5 to 7 days’ incubation period), misinterpretation of culture results (e.g., culture contamination) leading to underreporting, or both.
Pathogens | Incidence (%) |
---|---|
Gram-Positive Bacteria | |
Staphylococcus , coagulase-negative (e.g., S. epidermidis, S. capitis, S. hominis, S. warneri, S. lugdunensis, S. haemolyticus ) | 25–70 |
Staphylococcus aureus | 10–40 |
Streptococci (e.g., Streptococcus pyogenes , group B or C streptococci, Enterococcus, S. pneumoniae ) | 3–7 |
Propionibacterium spp. | Rare |
Corynebacterium spp. | 1–2 |
Gram-Negative Bacteria | |
Escherichia coli | 5–25 |
Klebsiella spp. | 5–10 |
Proteus spp. | 2–6 |
Pseudomonas spp. | 2–4 |
Acinetobacter spp. | 1–3 |
Other gram-negative bacteria (e.g., Neisseria spp., Haemophilus influenzae, Pasteurella ) | <1 |
Fungi | |
Candida spp. | <1 |
Histoplasma | <1 |
Cryptococcus | <1 |
Torulopsis | <1 |
Gram-negative bacteria (e.g., E. coli , Klebsiella spp., and Proteus spp . ) together are the cause of 5% to 25% of shunt-related infections. Pseudomonas spp. and Acinetobacter spp. are reported as well, but less frequently. Many other etiologic agents, including fungi (e.g., Candida, Histoplasma, Cryptococcus, Torulopsis ), Pasteurella multocida, Neisseria spp., Serratia marcescens, Listeria monocytogenes, nontuberculous mycobacteria, and others, have been reported less commonly as causing shunt-related infections. With the increase in the number of patients who are immunocompromised or have significant risk factors for infection (e.g., neutropenia, chronic intravenous catheters, prolonged administration of broad-spectrum antibiotics, and hyperalimentation), the incidence of these rare infections may increase. Bacteria that traditionally cause meningitis, such as Streptococcus pyogenes (group A strep), H. influenzae, S. pneumoniae, and Neisseria meningitidis, have been reported as causing shunt-related infections. Whether these cases were isolated shunt-related infections or extensions of meningitis into the ventricular system (i.e., ventriculitis) is not clear. Therefore if such bacteria are isolated from a suspected shunt and the patient has a communicating hydrocephalus, lumbar puncture should be performed to rule out meningitis. Of interest, most children with H. influenzae or S. pneumoniae infections recovered with antibiotic therapy alone and without shunt removal.
Pathogenesis
Several observations suggest that most CNS shunt–related infections are caused by inoculation of the organism during surgery or bacterial colonization during the early phase of wound healing. Contamination of the device by ward personnel during manipulation also was suggested. These observations include the facts that common skin flora (e.g., coagulase-negative staphylococci and S. aureus ) are the pathogens most frequently encountered, most of the infections occur within the first few weeks after surgery, and irrigation of the system is a risk factor for the development of infection. Another common mechanism (occurring with gram-negative bacterial infections) is retrograde progression of bacteria from the gastrointestinal tract (i.e., bowel perforation) or from the urinary tract (in the case of a ventriculoureteral shunt). Other mechanisms by which shunts become infected include (1) hematogenous infection in which a distant site of infection produces bacteremia leading to a shunt infection, although rare, and (2) wound or skin infection (e.g., cellulitis or decubitus ulcer) with direct extension from the infection site to the shunt.
The predominant role of coagulase-negative staphylococci and S. aureus in CNS shunt–related infection is the result of their being the major constituents of normal cutaneous flora, especially in young children, and their having the ability to adhere directly to the shunt (e.g., S. epidermidis ) or to host proteins covering the shunt (e.g., S. aureus ). In addition, coagulase-negative staphylococci (and some S. aureus strains) produce large amounts of extracellular slime (i.e., biofilm). More than 60% of staphylococci isolated from infected shunts produce biofilm. The biofilm of S. epidermidis is a mixture of teichoic acid and protein. Production of biofilm also was reported in corynebacterial infection , which may explain the increasing importance of this organism in CNS shunt–related infections. Biofilm facilitates attachment of these organisms to the surface of the shunt and protects the bacteria from the host immune defenses. Once the organisms are attached to the shunt material, they are extremely difficult to remove except by complete replacement of the shunt. In addition, penetration of antibiotic into the biofilm is variable, and the biofilm antagonizes the antimicrobial activity of some antibiotics, such as vancomycin. Moreover infections caused by nonadherent organisms were significantly more likely to be cured by antibiotics alone (without removal of the shunt) than were infections caused by adherent organisms. Similarly Diaz-Mitoma and colleagues found that both obstruction of ventriculoperitoneal shunts and failure to cure the infection with antibiotics alone occurred more frequently when infectious episodes were caused by biofilm-producing coagulase-negative staphylococci. Therefore complete removal of the shunt should be considered in patients with coagulase-negative staphylococci or S. aureus infection.
The immature humoral immune system of young infants is not likely to explain the increased incidence of shunt-related infections in patients younger than 6 months because these infants mount antistaphylococcal antibody responses that are comparable to those of older children. Although levels of immunoglobulins and complement proteins are lower in this young group, levels of these proteins normally are very low in the CSF of older individuals (CSF levels of IgG and IgA are between 0.25% and 0.5% of those in serum). In addition, the types of bacteria causing CNS shunt–related infections are not associated commonly with humoral immunodeficiency states, thus suggesting that humoral protection is less important in CNS shunt–related infections. Little is known about the possible role of reduced tissue immunity in these infections.
The foreign body nature of the shunt apparatus plays an important role in the local host defense defect. Electron-microscopic findings demonstrate irregularities in catheters, allowing microorganisms to be buried in the catheter. Other mechanisms that may contribute to shunt-related infections include (1) abnormal CSF flow (i.e., not being absorbed by the venous sinuses, thought to be important for prevention of infection in the CNS) and (2) interruption of the blood–brain barrier by the shunt catheter, with the creation of a direct tract between the subcutaneous tissues and the ventricles resulting in significant compromise in host defenses.
Clinical Manifestations
The initial signs and symptoms of most patients with shunt-related infections are nonspecific and include mild to moderate fever, malaise, irritability, nausea, vomiting, vague abdominal pain, and headache. With such nonspecific findings, the physician must be careful to differentiate between a potential shunt-related infection and an intercurrent viral or bacterial infection of the upper respiratory, urinary, or gastrointestinal tract. Examination of the CSF (from a shunt tap) may be helpful. Only a minority of patients have the classic signs and symptoms of CNS inflammation, such as a stiff neck, bulging fontanelle, change in mental status, cranial nerve palsy, or papilledema. In some patients the shunt tract may be infected, with evidence of cellulitis, dehiscence, or both of the surgical wounds. Tenderness, edema, or erythema along the tract itself may be the only sign. Patients with known seizures frequently develop seizures with shunt malfunction (inducing in cases of infection).
The type of shunt affects the nature of the infection. For example, ventriculoperitoneal shunt–related infections may cause symptoms and signs confined to the abdominal cavity, such as abdominal pain, tenderness (with or without guarding), intestinal obstruction, or spontaneous bacterial peritonitis. Rarely a distal shunt-related infection will be manifested as frank ascites as a result of CSF malabsorption. A relatively common complication of ventriculoperitoneal shunts is an inflammatory peritoneal exudate that may lead to CSF loculation and the subsequent formation of a peritoneal pseudocyst. These pseudocysts often are palpable and can be visualized by ultrasonography or CT. Bacteria are isolated in a third of cases, suggesting that infection may play a role in the pathogenesis of pseudocysts. In most cases, however, a high index of suspicion is required because the initial abdominal symptoms occur with no other signs of shunt malfunction.
A unique complication of patients with ventriculoatrial shunts is the development of immune complex disease such as shunt nephritis (a form of acute glomerulonephritis), arthritis, or rash. In most of these cases, the infecting organism is S. epidermidis, but other bacteria such as Corynebacterium can cause this complication. In the case of shunt nephritis, the patient has fever, edema, malaise, hepatosplenomegaly, hypocomplementemia, anemia, azotemia, hematuria, and proteinuria. Pathologic findings consist of mesangial hypercellularity and granular deposits of immunoglobulins and complement along the glomerular membrane. Rarely arthritis may be the initial symptom.
Diagnosis
Although shunt-related infections are not common, the nonspecific signs and symptoms and the insidious onset in many cases render establishing a diagnosis difficult. Therefore in any patient with a CNS shunt and fever without an obvious source, a shunt-related infection should be considered, especially in the case of persistent symptoms. A higher index of suspicion for shunt-related infection is needed in young patients with new fever within 3 to 6 months after shunt placement. The only definitive diagnostic test is direct staining and culture of CSF. Tapping the shunt or sampling fluid in direct contact with the shunt should be performed if no signs or symptoms of increased intracranial pressure are noted. CNS imaging (CT or MRI) is recommended before the tap is done if such symptoms exist. The shunt tap should be performed with careful sterile technique by a practitioner familiar with the technique and underlying hardware.
When percutaneous needle shunt aspiration is performed, the area around the shunt valve or reservoir should be sterilized and butterfly needle inserted. Measurement of opening pressure can help diagnose a distal malfunction (increased pressure) or proximal shunt obstruction (less than expected pressure). Gentle aspiration of CSF sometimes is performed if no fluid returns spontaneously. If only a few drops of CSF can be obtained, the more important tests, Gram stain and culture, should be performed first.
CSF should be tested for glucose concentration, differential cell count, Gram stain, and culture. Protein concentration is requested often but is of very limited help in evaluating the presence or absence of an infection because high protein levels are found in many patients with shunt malfunction and no infection. In contrast, normal protein levels have been reported in many patients with shunt-related infections. A low glucose level suggests an infection, although this is not sensitive for shunt-related infection. Typically CSF pleocytosis with a predominance of PMNs is indicative of a shunt-related infection. A count of 100 cells/µL or more is highly suggestive of infection. According to one study, a CSF WBC count greater than 100 cells/µL with more than 10% PMN cells in febrile patients is 82% sensitive and 99% specific for shunt infection, with a positive predictive value of 93%. In contrast, the negative predictive value is 95% for a sample with less than 10% PMNs. A recent review suggests that infection with gram-negative bacteria resulted in a higher initial and peak WBC count, with a greater percentage of PMNs than gram-positive bacteria. In some cases the finding of a positive CSF culture is interpreted as a shunt-related infection despite a normal WBC count (<10 WBCs/mm 3 ) and the absence of clinical symptoms, when the positive culture probably represents colonization or contamination. Other cells, such as mononuclear cells or eosinophils can also predominate during an infection. If eosinophilia is the predominant cellular response, an allergy to the shunt (e.g., silicone ) or the materials used for sterilization (e.g., ethylene oxide ) or use of an antibiotic-impregnated catheter or intraventricular administration of antibiotics (e.g., gentamicin and vancomycin ) should be considered. It is important to note that eosinophilia (without these causes, including infection) is relatively common (but transient) in children with a CNS shunt.
Interpretation of the WBC count should be done cautiously if the red blood cell count is high because the increased number of WBCs can be the result of blood spilling into the CSF or be part of the inflammatory response to the presence of blood (i.e., chemical ventriculitis). A negative Gram stain result does not exclude an infection, and one should wait for the results of culture. Ventricular fluid always should be cultured anaerobically as well as aerobically. Although most bacteria causing shunt-related infections grow within 48 to 78 hours, cultures should be held for 7 days (if still negative) for fastidious organisms such as Propionibacterium. The possibility of contamination or colonization of the shunt without infection should be considered when the culture is positive but other CSF parameters are normal. If such a scenario occurs and bacteria are growing only from one sample (e.g., a shunt tap) and not from subsequent CSF cultures, a shorter course of therapy may be sufficient.
Blood cultures, a peripheral complete blood count (CBC), and ultrasound of the abdomen (for ventriculoperitoneal shunts) are of limited value. For example, although 90% of patients with ventriculoatrial shunt–related infection will have a positive blood culture, fewer than 10% of patients with other shunt-related infections will have a positive culture. In more than a third of patients with shunt-related infections, no elevation in the peripheral WBC count was found. Some investigators suggest that blood CRP levels may be helpful in establishing the diagnosis of shunt-related infection when other concurrent infections (e.g., sinusitis, pneumonia) were excluded. The triad of fever, abnormal CSF WBC count, and greater than 5% eosinophilia is highly predictive of a shunt-related infection.
Treatment
A variety of medical and surgical approaches to treatment of an infected shunt have been suggested. Regimens include (1) the use of antibiotics (systemically, with or without intraventricular administration) without removal of the shunt hardware; (2) removal of the infected shunt followed by immediate insertion of a new shunt and the administration of antibiotics ; (3) removal of the infected shunt and insertion of an extraventricular device (EVD) to monitor the patient’s response to the accompanying antibiotic therapy, with a new shunt inserted only when the ventricular system is sterilized; (4) removal of the infected shunt followed by a stereotactic third ventriculostomy and administration of antibiotics; and (5) externalization of only the distal (e.g., peritoneal) catheter, along with the administration antibiotics.
The use of antibiotics alone without surgery was justified by the need to maintain CSF drainage and avoid costly operations and lengthy duration of hospital stay. The low success rate of this approach (33%) and the higher mortality rate associated with it suggest that it should not be used ( Table 74.2 ). Of interest, the failure rate was much higher with infections caused by biofilm-producing organisms than with infections caused by bacteria that do not produce biofilm. Only in shunted patients with purulent meningitis caused by S. pneumoniae, N. meningitidis, or H. influenzae did the administration of systemic antibiotics alone without removal of the shunt seem to be an effective option.
Study | Antibiotics Alone a | Antibiotics and Immediate Replacement With New Shunt | Antibiotics, Removal of Shunt, and Insertion of EVD |
---|---|---|---|
Schoenbaum | 5/30 b | 25/26 | |
Nelson | 10/13 | 46/46 | |
Salmon | 5/10 | ||
Sells | 1/8 | 1/6 | 9/9 |
James | 3/10 | 9/10 | |
Venes | 6/9 | 3/3 | |
James | 4/11 | 11/13 | 21/22 |
Wald | 15/20 | ||
Mates | 7/8 | ||
Shurtleff | 2/27 | 6/20 | 19/19 |
Morrice | 4/14 | 19/23 | 14/18 |
Frame | 8/11 | 21/27 | |
Forward | 8/15 | 2/2 | 13/13 |
Luthardt | 1/17 | ||
O’Brien | 11/11 | 15/19 | 9/9 |
Walters | 13/92 | 11/21 | 44/71 |
Swayne | 19/20 | ||
Ronan | 3/4 | 4/7 | 21/22 |
Stamos | 23/23 | ||
Younger | 4/11 | 42/46 | |
Total (success rate) | 99/302 (32.8%) | 143/203 (70.4%) | 275/311 (88.4%) |
Combining immediate replacement of the infected shunt with a new shunt and antibiotic therapy has a higher rate of success (70%; see Table 74.2 ) than does the use of antibiotics alone. Nonetheless it is less effective than removal of the infected shunt accompanied by insertion of an EVD and administration of antibiotic therapy (88% success rate; see Table 74.2 ). A decision analysis of 17 studies reached the same conclusion and suggested that “this treatment option has the highest cure rate and the lowest failure and mortality rates.” In addition, lack of the ability to monitor when the ventricular fluid is sterilized results in a longer period of systemic antibiotic therapy (e.g., 4 to 8 weeks) and may lead to an increase in iatrogenic infections and cost.
For infection that involves only the distal part of the shunt (e.g., pseudocyst, appendicitis, erythema or swelling along the shunt tract, or surgical wound infection), externalization of only the distal end of the shunt along with the administration of antibiotic therapy is recommended by some neurosurgeons. Potential advantages of this technique include (1) diversion of CSF from an infected area to avoid ascending infection, (2) maintenance of CSF flow to prevent increased intracranial pressure (ICP), (3) the ability to perform frequent CSF sampling, and (4) the capability of monitoring therapy. The disadvantage is that early infection or colonization of the proximal portion of the shunt may be obscured by the antibiotic treatment and become active after discontinuation of therapy and reinsertion of the distal part. In patients with P. acne –related distal shunt infection, catheter removal without (or very short) antibiotic treatment is sufficient.
Internal shunting by a third ventriculostomy with avoidance of a prosthetic device during the infected CSF shunt removal was investigated as another option to reduce reinfection. Although this procedure was shown to be effective in managing patients with refractory shunt-related infections who have a noncommunicating hydrocephalus, patent subarachnoid space, and adequate CSF absorption, the prevention of reinfection was marginal. Yet it seems that the reinserted ventriculoperitoneal shunts after a third ventriculostomy have a better longevity than those inserted without this procedure. Shunt independence for extended periods was documented in patients without myelomeningocele. The success rate is lower in those with myelomeningocele or hemorrhage or after meningitis.
The most effective treatment of shunt-related infections is to remove the entire infected shunt and insert an EVD to control ICP and monitor the infection. After antibiotic therapy has been successful, a new shunt is placed. With this approach, treatment success is very high (see Table 74.2 ), with more rapid clearance of the infection and a shorter duration of therapy.
The choice of intravenous antibiotic depends on local patterns of antimicrobial susceptibility and the ability of the antibiotic to penetrate the blood-brain barrier. With the notable rate of methicillin-resistant staphylococci, vancomycin should be used as initial therapy while awaiting bacteriologic identification and the results of antibiotic sensitivity testing. Effort should be made to discontinue vancomycin promptly following proof that organism is sensitive to the semisynthetic penicillins. In addition, despite in vitro data that bacteria are highly sensitive to first-generation cephalosporins (e.g., cephalothin, cefazolin), they should not be used due to poor penetration of the blood-brain barrier. Linezolid has been used successfully in cases of shunt infection. This drug has a good CSF penetration, with concentrations above the minimal inhibitory concentration (MIC) of most gram-positive bacteria, including MRSA. The limited data available suggest that linezolid should be considered cautiously when vancomycin fails or cannot be given. Rifampin should be considered as one of the drugs in the combination for gram-positive bacteria for three reasons. First, most staphylococci are still sensitive to this antibiotic and their MIC is significantly lower than that of other antistaphylococcal drugs. Unfortunately the use of rifampin alone may be followed rapidly by rifampin-resistant variants. Second, rifampin penetrates CSF well and easily achieves a greater than 10-fold level over the MIC of most staphylococci. Third, rifampin has demonstrated good bactericidal activity even when staphylococci were embedded in biofilm. In contrast, staphylococcal biofilm inhibits the antimicrobial activity of vancomycin.
To achieve more consistent and efficient eradication of bacteria, the CSF drug level should be at least 10 times higher than the MIC of the pathogen. Therefore the dosage of antibiotic or antibiotics and the dosing interval should be maximized (i.e., “meningeal schedule”). If the selected antibiotic does not clear the infection within 2 to 3 days and no improvement occurs in CSF biochemical and WBC parameters (i.e., seemingly inadequate control of the infection), measurement of the bactericidal titer of ventricular fluid should be considered. To determine bactericidal titer, 1 mL of CSF (if CSF production is >5/mL/h) at the expected peak antibiotic level (i.e., 2 to 3 hours after the antibiotic is given parenterally) is diluted serially with culture media to produce dilutions of 1 : 2, 1 : 4, 1 : 8, and 1 : 16. To these dilutions, an equal amount of medium with 10 5 colony forming units/mL of the offending bacteria is added (the final CSF dilution is 1 : 4, 1 : 8, 1 : 16, and 1 : 32). After 24 hours of incubation, the tubes are observed for turbidity, which reflects the growth of bacteria. If no turbidity is seen in the 1 : 8 and 1 : 16 or higher dilution tubes, the CSF level of the antibiotic probably is sufficient, and continued growth of bacteria from CSF may be caused by colonization of the EVD or contamination. On the other hand, if turbidity is noted in the tube with less than a 1 : 8 dilution (i.e., a 1 : 4 dilution), the CSF level of antibiotic may not be sufficient to combat the infection and the addition of another antibiotic or change to a different antibiotic is warranted.
Selection of the initial antibiotic before culture results are known can be based on the patient’s clinical and CSF findings. The initial diagnostic step after empiric antibiotic therapy is evaluation of the CSF Gram stain. If the Gram stain result is positive for gram-positive bacteria (e.g., Staphylococcus or Streptococcus ), the drug of choice is vancomycin. If the Gram stain shows gram-negative bacteria, the drug of choice is cefotaxime (or ceftriaxone) or, in cases of suspected pseudomonas or multidrug-resistant infection, meropenem. Treatment with an aminoglycoside is acceptable, but the outcome seems to be less favorable because of poorer penetration into CSF. If the Gram stain result is negative, other CSF parameters (e.g., WBC count and glucose) should be examined. If either is abnormal and the patient is not severely sick, vancomycin alone may be considered because the chance of having a gram-negative infection is low.
In contrast, if the patient is more ill, coverage for gram-negative bacteria should be added (e.g., cefotaxime or ceftriaxone). In nontoxic patients with normal CSF parameters and no distal symptoms (e.g., peritonitis or wound or tract infection), antibiotic therapy can be withheld until the results of culture are known. On the other hand, if distal signs or symptoms exist, therapy is tailored according to the site. In patients with skin involvement, vancomycin is the drug of choice, whereas in febrile patients with abdominal symptoms, the combination of cefotaxime (or ceftriaxone) and metronidazole (to cover anaerobic bacteria) is preferred. An alternative for gram-negative plus anaerobic coverage in a seriously ill patient with history of broad spectrum antibiotic exposure or multidrug-resistant organism is meropenem.
Direct instillation of antibiotics into the ventricular system to increase their levels is suggested by some experts. Such treatment may be considered in patients with persistently positive cultures despite intravenous therapy or multidrug-resistant organisms requiring specific antibiotics with inadequate CSF penetration. Several studies have reported promising results using combination of intravenous and intraventricular therapy. Unfortunately the suggested doses for intraventricular treatment have been determined empirically on only a small number of patients, and their pharmacokinetics and pharmacodynamics have not been studied well. This therapy is not without risk, especially when the recommended doses often are much higher than those found to cause neurotoxicity. Pleocytosis and eosinophilia also have occurred in patients receiving intraventricular vancomycin or gentamicin. In addition, preservative-containing preparations should be checked for appropriateness for intraventricular instillation. Limited pharmacokinetic data suggest that clearance of the instilled intraventricular antibiotic is sufficiently slow to allow once-daily administration. If possible, the EVD should be closed for 30 to 60 minutes after the drug is administered. If the EVD cannot be closed and the amount of CSF drainage exceeds 7 to 10 mL/h, the frequency of intraventricular antibiotic instillation should be increased to twice daily.
Antibiotics commonly recommended for intravenous and intraventricular use according to the etiologic agent are shown in Table 74.3 , although antibiotic choice should be based on antimicrobial susceptibility results of the isolated pathogen. A small study in adults showed that administration of vancomycin 10 mg intraventricularly once daily was safe and achieved levels greater than 5 µg/mL (the recommended CSF trough level needed to achieve cure) for up to 21 hours. Because of the potential toxicity of the empirically recommended intraventricular antibiotic doses and the unpredictability of CSF levels, irrigation of the ventricular system with a known concentration of an antibiotic solution is preferred when systemic antibiotics fail to eradicate the bacteria. To achieve irrigation, two EVDs must be inserted to produce a continuous flow of solution. The concentration of antibiotic in the solution should be equal to the highest safe plasma level when the drug is given intravenously. For example, for treating gram-negative bacteria sensitive to amikacin, amikacin at a dose of 30 to 40 mg/L of saline solution (producing a concentration of 30–40 µg/mL) is recommended. Gentamicin 10 to 12 mg/L (using a special intrathecal preparation) is an acceptable alternative when the gentamicin MIC for the bacteria is less than 1 µg/mL. The antibiotic solution is administered through one EVD at a rate of 10 mL/h, and the second EVD is left open to drain the fluid and to remove debris and pus from the ventricles.
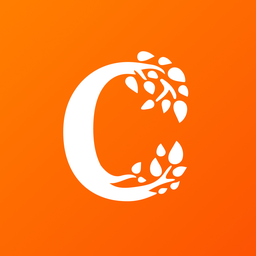
Full access? Get Clinical Tree
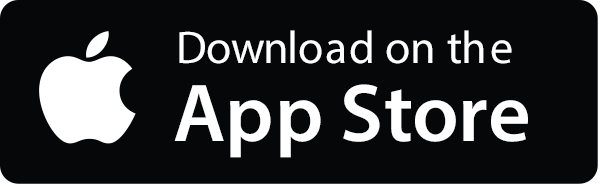
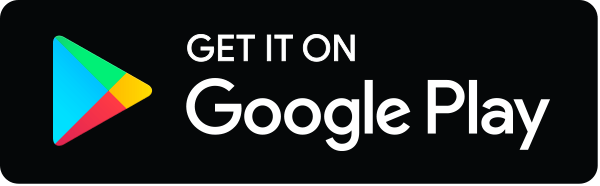