Background
Late-onset fetal growth restriction (FGR) is often undetected prior to birth, which puts the fetus at increased risk of adverse perinatal outcomes including stillbirth.
Objective
Measuring RNA circulating in the maternal blood may provide a noninvasive insight into placental function. We examined whether measuring RNA in the maternal blood at 26-30 weeks’ gestation can identify pregnancies at risk of late-onset FGR. We focused on RNA highly expressed in placenta, which we termed “placental-specific genes.”
Study Design
This was a case-control study nested within a prospective cohort of 600 women recruited at 26-30 weeks’ gestation. The circulating placental transcriptome in maternal blood was compared between women with late-onset FGR (<5th centile at >36+6 weeks) and gestation-matched well-grown controls (20-95th centile) using microarray (n = 12). TaqMan low-density arrays, reverse transcription-polymerase chain reaction (PCR), and digital PCR were used to validate the microarray findings (FGR n = 40, controls n = 80).
Results
Forty women developed late-onset FGR (birthweight 2574 ± 338 g, 2nd centile) and were matched to 80 well-grown controls (birthweight 3415 ± 339 g, 53rd centile, P < .05). Operative delivery and neonatal admission were higher in the FGR cohort (45% vs 23%, P < .05). Messenger RNA coding 137 placental-specific genes was detected in the maternal blood and 37 were differentially expressed in late-onset FGR. Seven were significantly dysregulated with PCR validation ( P < .05). Activating transcription factor-3 messenger RNA transcripts were the most promising single biomarker at 26-30 weeks: they were increased in fetuses destined to be born FGR at term (2.1-fold vs well grown at term, P < .001) and correlated with the severity of FGR. Combining biomarkers improved prediction of severe late-onset FGR (area under the curve, 0.88; 95% CI 0.80-0.97). A multimarker gene expression score had a sensitivity of 79%, a specificity of 88%, and a positive likelihood ratio of 6.2 for subsequent delivery of a baby <3rd centile at term.
Conclusion
A unique placental transcriptome is detectable in maternal blood at 26-30 weeks’ gestation in pregnancies destined to develop late-onset FGR. Circulating placental RNA may therefore be a promising noninvasive test to identify pregnancies at risk of developing FGR at term.
Introduction
Fetal growth restriction (FGR) is the single biggest risk factor for stillbirth. Survivors of FGR are at increased risk of permanent neurodevelopmental, cardiovascular, and metabolic morbidity that persists into adulthood. FGR affects 5-10% of pregnancies and may occur at any stage in pregnancy, but is considered late onset when it occurs >34-36 weeks’ gestation. Recognition of FGR, antenatal surveillance, and timely delivery reduces the risk of adverse perinatal outcomes and long-term health sequelae. Unfortunately, detecting late-onset FGR is challenging, as no effective test exists, and the majority of babies (85%) with late-onset FGR are unrecognized prior to birth.
Improving the ability to detect growth-restricted pregnancies using combined first- and second-trimester blood-based biomarkers and imaging techniques has been identified as a research priority to improve perinatal outcomes. While these tests have a limited efficacy to detect early-onset FGR, they are very poor predictors of term outcomes. The standard approach to identifying late-onset FGR is serial abdominal palpation and measurement of the fundal height at each antenatal visit, but this technique identifies <30% of pregnancies at risk of FGR, with an 88% specificity. Routine third-trimester ultrasound has also failed to accurately predict all cases of late-onset FGR and its use has not been shown to improve perinatal outcomes.
Placental dysfunction is the causative mechanism of the majority of FGR in developed countries. However, some babies born small are not pathologically growth restricted but small for gestational age (SGA) and healthy. Prior to 36 weeks, it is possible to differentiate FGR and SGA by interrogating fetal Doppler. In contrast, this performs poorly at identifying late-onset FGR, where the umbilical artery Doppler is likely to be normal even in the presence of severe FGR. Therefore, blood-based biomarkers that can identify deteriorating placental function may help to identify pregnancies at risk of adverse outcomes. Multiple protein biomarkers have been proposed for this use but none have sufficient accuracy to be recommended for clinical practice. Biomarkers of abnormal angiogenesis, placental growth factor and soluble fms-like tyrosine kinase-1, measured in the third trimester are predictive of preeclampsia but perform less well at predicting late-onset FGR.
Nucleic acids are released from the placenta into the maternal blood during pregnancy, a finding that has been exploited for detection of fetal aneuploidy. In addition to cell-free DNA, RNA from the placenta also circulates in the maternal blood and offers a novel way to monitor placental function. We have previously identified RNA transcripts highly expressed in the placenta, relative to other tissues, that may therefore be ideal biomarkers of placenta function and we have termed these “placental-specific genes” (PSGs). We have shown that these circulating placental RNAs are differentially expressed in pregnancies complicated by early-onset FGR and preeclampsia, and can be used to detect pregnancies complicated by early-onset FGR. We hypothesized that circulating placental RNA would also be differentially expressed in pregnancies at risk of late-onset FGR, and that these changes would be detectable from early in the third trimester. We therefore investigated whether the expression of placental RNA in the maternal blood at 26-30 weeks’ gestation could discriminate which pregnancies were destined to develop late-onset FGR.
Materials and Methods
Study participants and specimens
A nested case-control study was performed from a larger prospective study, which recruited 600 consecutive women between 26-30 weeks’ gestation at Monash Medical Center (a single large, tertiary maternity center in Melbourne, Australia) from 2009 through 2011. Women were recruited at the time of gestational diabetes screening, with an additional whole blood sample collected at the same time as the fasting sample.
Women continued with routine antenatal and intrapartum care as determined by their treating obstetrician or maternity care provider. After delivery, pertinent demographic, clinical, and obstetric data were collected from the medical records. Late-onset FGR was defined as women delivering a baby <5th centile at >36 completed weeks’ gestation. Severe FGR was defined as a baby <3rd centile. Delivery for suspected intrapartum compromise was based on the obstetrician’s documentation regarding abnormal fetal heart rate patterns, abnormal intrapartum fetal blood sampling, or both. All women were normotensive at inclusion. Women who developed gestational hypertension (blood pressure >140/90 mm Hg) were included but women with preeclampsia (proteinuria or biochemical abnormalities) were excluded. Multiple pregnancies and known fetal anomalies were excluded. Controls were matched in a 2:1 ratio according to parity, ethnicity, gestational age at blood sampling, and timing of sampling within 3 months of case, but delivered a baby between the 20-95th centile at term. Birthweight centiles were calculated using Australian national birthweight standards. All women provided written informed consent and the study protocol was approved by the Southern Health Research Ethics Committee (09019B).
Sample collection
A total of 2.5 mL of maternal peripheral whole blood was collected in PAXgene whole blood RNA tubes (PreAnalytix, Hombrechtikon, Switzerland) at the time of the fasting glucose test for diabetes screening. They were stored at room temperature for 24 hours, then at –80°C until processing.
RNA preparation
Total RNA was extracted using the PAXgene microRNA system (PreAnalytix, Hombrechtikon, Switzerland) according to manufacturer’s instructions. Genomic DNA was removed using deoxyribonuclease treatment. Total RNA was eluted and stored at –80°C if not used immediately. RNA concentration and purity was measured using a NanoDrop ND100 spectrophotometer (Thermo Scientific, Pittsburgh, PA). For microarray, sample quality was evaluated further by the BioAnalyzer 2100 system (Agilent, Santa Clara, CA).
Microarray analyses
RNA samples from cases (n = 12) and controls (n = 12) were hybridized to HumanHT-12 Expression BeadChips (Illumina Inc, San Diego, CA). BeadChip processing was performed by the Australian Genome Research Facility (Melbourne, Australia) according to manufacturer’s instructions. Scanned images were analyzed using Illumina GenomeStudio. Gene expression analysis was performed using BioConductor in R ( www.r-project.org ) after quality control, preprocessing, background subtraction, and normalization was performed. Linear modeling was performed using the Limma package and fold change calculated by the t test adjusted for multiple comparisons using the Benjamini and Hochberg methodology for false discovery rate. GSEA software ( www.broadinstitute.org/gsea ) was used to investigate overrepresentation of biological pathways, including the 137 PSG set developed in our previous study.
Validation with TaqMan low-density array, and with reverse-transcription and digital polymerase chain reaction analysis
TaqMan low-density array (TLDA) allows validation of a large number of genes in a single experiment and is fully quantitative. Therefore we performed initial validation of the microarray using customized microfluidic cards: TLDA (Applied Biosystems, Foster City, CA). Each TLDA contained a set of TaqMan gene expression assays for 45 placental genes and 3 internal controls (glyceraldehyde-3-phosphate dehydrogenase, beta-2-microglobulin, and glucuronidase beta). For each sample 200 ng of total RNA was reverse transcribed using random hexamers with Superscript Vilo reverse transcriptase (Invitrogen, Carlsbad, CA). A total of 100 μL reaction mixture containing 50 μL complementary DNA (cDNA) template in RNase-free water and an equal volume of TaqMan Universal Master Mix (Applied Biosystems) was added to each TLDA fill reservoir. One reservoir per sample was filled. After sealing the plate, it was run on a 7900HT real-time instrument (Applied Biosystems).
While microarray and TLDA are effective screening tools for multiple biomarkers, to develop a potential biomarker the findings were further validated using individual polymerase chain reaction (PCR) for each placental transcript. Reverse transcription-polymerase chain reaction (RT-PCR) is the conventional methodology for quantification RNA transcripts but relies on the stable expression of housekeeping genes in all samples. Digital PCR (dPCR) has the advantage over RT-PCR in that it quantifies the copy number of each transcript, has greater precision, and does not require the use of housekeeping genes. Therefore we performed RT-PCR and dPCR to validate the findings of the TLDA and develop a potential biomarker for prediction of late-onset FGR.
Real-time RT-PCR validation of the dysregulated placental genes from the TLDA were then performed using the same TaqMan gene expression assays. All RT-PCR were performed in triplicate using multiple negative controls including RT and no-template controls. We chose the combined expression of glyceraldehyde-3-phosphate dehydrogenase, beta-2-microglobulin, and glucuronidase beta as our internal control having previously validated that their expression was not altered in these samples. The comparative cycle threshold method was used to determine relative expression. Nonparametric statistical tests were used for comparison of gene expression and the Bonferroni correction for multiple comparisons was used where appropriate.
dPCR was performed using the QuantStudio 3D PCR platform (Applied Biosystems) using TaqMan primers and probes. dPCR reports absolute transcript copy number. Utilizing nanofluidic chips containing 20,000 partitions, dPCR does not require normalization to housekeeping genes and allows increased precision through use of multiple replicates, therefore providing more accurate quantitation than conventional RT-PCR.
Clinical data and validation analyses
All clinical and PCR data were statistically analyzed using software (GraphPad Prism, v 5; GraphPad Software Inc, San Diego, CA). Differences in RT-PCR gene expression was assessed using Mann-Whitney U or Kruskal–Wallis test, or where the data were considered normally distributed, the t test or analysis of variance. Patient characteristics were compared using χ 2 where appropriate. Significance was defined as P < .05.
Materials and Methods
Study participants and specimens
A nested case-control study was performed from a larger prospective study, which recruited 600 consecutive women between 26-30 weeks’ gestation at Monash Medical Center (a single large, tertiary maternity center in Melbourne, Australia) from 2009 through 2011. Women were recruited at the time of gestational diabetes screening, with an additional whole blood sample collected at the same time as the fasting sample.
Women continued with routine antenatal and intrapartum care as determined by their treating obstetrician or maternity care provider. After delivery, pertinent demographic, clinical, and obstetric data were collected from the medical records. Late-onset FGR was defined as women delivering a baby <5th centile at >36 completed weeks’ gestation. Severe FGR was defined as a baby <3rd centile. Delivery for suspected intrapartum compromise was based on the obstetrician’s documentation regarding abnormal fetal heart rate patterns, abnormal intrapartum fetal blood sampling, or both. All women were normotensive at inclusion. Women who developed gestational hypertension (blood pressure >140/90 mm Hg) were included but women with preeclampsia (proteinuria or biochemical abnormalities) were excluded. Multiple pregnancies and known fetal anomalies were excluded. Controls were matched in a 2:1 ratio according to parity, ethnicity, gestational age at blood sampling, and timing of sampling within 3 months of case, but delivered a baby between the 20-95th centile at term. Birthweight centiles were calculated using Australian national birthweight standards. All women provided written informed consent and the study protocol was approved by the Southern Health Research Ethics Committee (09019B).
Sample collection
A total of 2.5 mL of maternal peripheral whole blood was collected in PAXgene whole blood RNA tubes (PreAnalytix, Hombrechtikon, Switzerland) at the time of the fasting glucose test for diabetes screening. They were stored at room temperature for 24 hours, then at –80°C until processing.
RNA preparation
Total RNA was extracted using the PAXgene microRNA system (PreAnalytix, Hombrechtikon, Switzerland) according to manufacturer’s instructions. Genomic DNA was removed using deoxyribonuclease treatment. Total RNA was eluted and stored at –80°C if not used immediately. RNA concentration and purity was measured using a NanoDrop ND100 spectrophotometer (Thermo Scientific, Pittsburgh, PA). For microarray, sample quality was evaluated further by the BioAnalyzer 2100 system (Agilent, Santa Clara, CA).
Microarray analyses
RNA samples from cases (n = 12) and controls (n = 12) were hybridized to HumanHT-12 Expression BeadChips (Illumina Inc, San Diego, CA). BeadChip processing was performed by the Australian Genome Research Facility (Melbourne, Australia) according to manufacturer’s instructions. Scanned images were analyzed using Illumina GenomeStudio. Gene expression analysis was performed using BioConductor in R ( www.r-project.org ) after quality control, preprocessing, background subtraction, and normalization was performed. Linear modeling was performed using the Limma package and fold change calculated by the t test adjusted for multiple comparisons using the Benjamini and Hochberg methodology for false discovery rate. GSEA software ( www.broadinstitute.org/gsea ) was used to investigate overrepresentation of biological pathways, including the 137 PSG set developed in our previous study.
Validation with TaqMan low-density array, and with reverse-transcription and digital polymerase chain reaction analysis
TaqMan low-density array (TLDA) allows validation of a large number of genes in a single experiment and is fully quantitative. Therefore we performed initial validation of the microarray using customized microfluidic cards: TLDA (Applied Biosystems, Foster City, CA). Each TLDA contained a set of TaqMan gene expression assays for 45 placental genes and 3 internal controls (glyceraldehyde-3-phosphate dehydrogenase, beta-2-microglobulin, and glucuronidase beta). For each sample 200 ng of total RNA was reverse transcribed using random hexamers with Superscript Vilo reverse transcriptase (Invitrogen, Carlsbad, CA). A total of 100 μL reaction mixture containing 50 μL complementary DNA (cDNA) template in RNase-free water and an equal volume of TaqMan Universal Master Mix (Applied Biosystems) was added to each TLDA fill reservoir. One reservoir per sample was filled. After sealing the plate, it was run on a 7900HT real-time instrument (Applied Biosystems).
While microarray and TLDA are effective screening tools for multiple biomarkers, to develop a potential biomarker the findings were further validated using individual polymerase chain reaction (PCR) for each placental transcript. Reverse transcription-polymerase chain reaction (RT-PCR) is the conventional methodology for quantification RNA transcripts but relies on the stable expression of housekeeping genes in all samples. Digital PCR (dPCR) has the advantage over RT-PCR in that it quantifies the copy number of each transcript, has greater precision, and does not require the use of housekeeping genes. Therefore we performed RT-PCR and dPCR to validate the findings of the TLDA and develop a potential biomarker for prediction of late-onset FGR.
Real-time RT-PCR validation of the dysregulated placental genes from the TLDA were then performed using the same TaqMan gene expression assays. All RT-PCR were performed in triplicate using multiple negative controls including RT and no-template controls. We chose the combined expression of glyceraldehyde-3-phosphate dehydrogenase, beta-2-microglobulin, and glucuronidase beta as our internal control having previously validated that their expression was not altered in these samples. The comparative cycle threshold method was used to determine relative expression. Nonparametric statistical tests were used for comparison of gene expression and the Bonferroni correction for multiple comparisons was used where appropriate.
dPCR was performed using the QuantStudio 3D PCR platform (Applied Biosystems) using TaqMan primers and probes. dPCR reports absolute transcript copy number. Utilizing nanofluidic chips containing 20,000 partitions, dPCR does not require normalization to housekeeping genes and allows increased precision through use of multiple replicates, therefore providing more accurate quantitation than conventional RT-PCR.
Clinical data and validation analyses
All clinical and PCR data were statistically analyzed using software (GraphPad Prism, v 5; GraphPad Software Inc, San Diego, CA). Differences in RT-PCR gene expression was assessed using Mann-Whitney U or Kruskal–Wallis test, or where the data were considered normally distributed, the t test or analysis of variance. Patient characteristics were compared using χ 2 where appropriate. Significance was defined as P < .05.
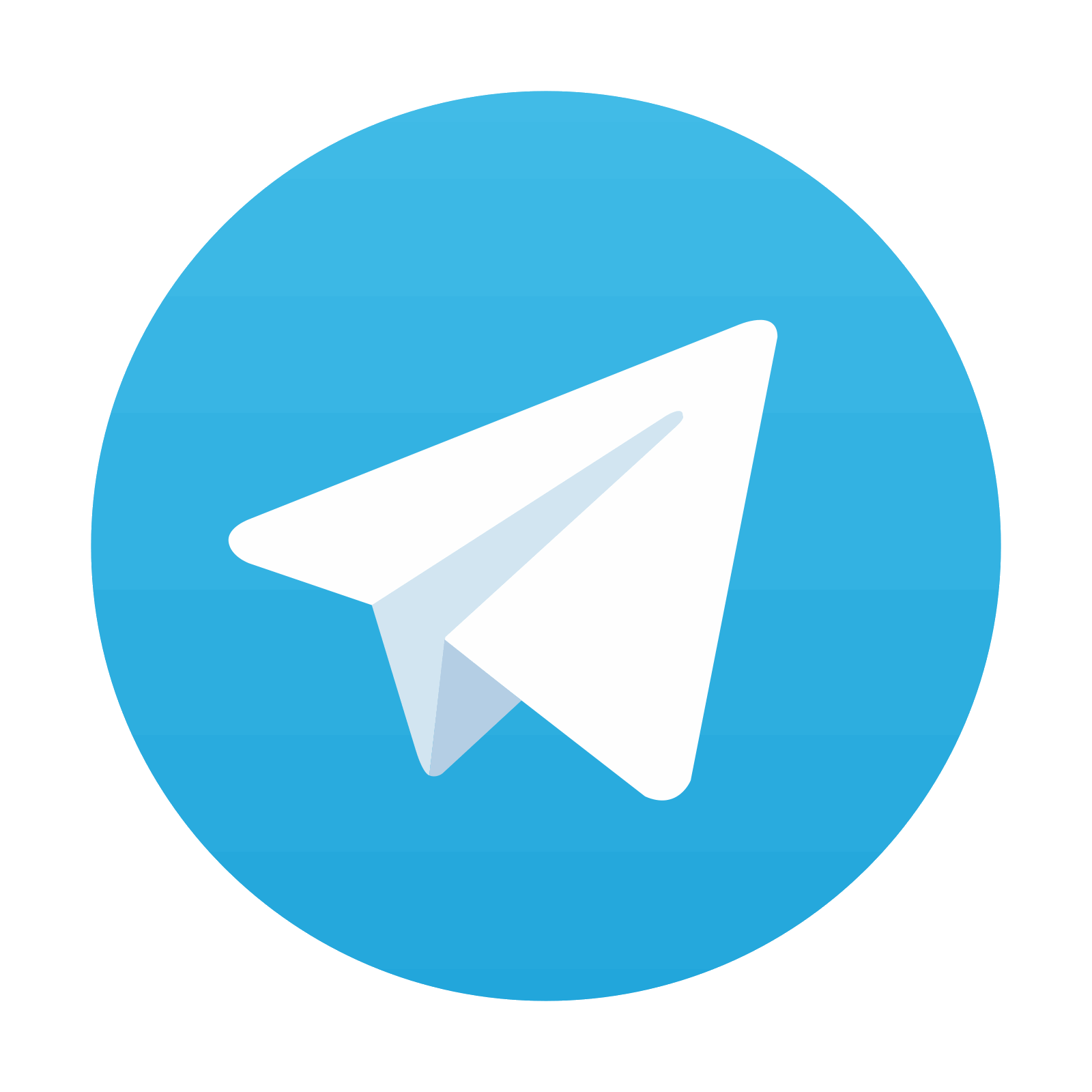
Stay updated, free articles. Join our Telegram channel
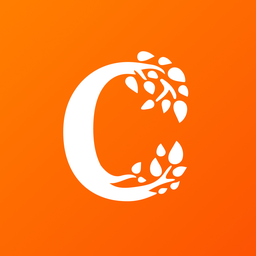
Full access? Get Clinical Tree
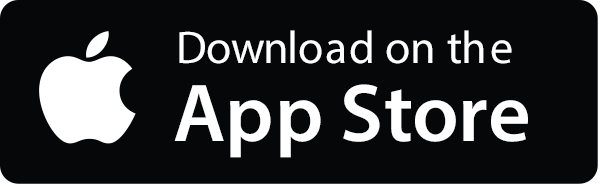
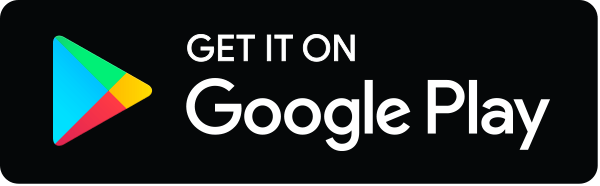