BACKGROUND
Hypoxia and hypoxemia are common reasons for hospital admission, usually in the context of a primary respiratory illness1. An understanding of the evaluation, diagnosis, and monitoring of patients with hypoxemia can improve patient care and resource utilization.
The process of delivering oxygen (O2) to tissues is complex, but knowledge of this process facilitates an understanding of the clinical aspects of hypoxemia and hypoxia. Providing adequate tissue oxygenation involves the pulmonary, cardiovascular, and hematology systems and is hampered by the relatively slow tissue diffusion of O2. O2 always moves down its partial pressure gradient from dry atmospheric air (partial pressure of O2 [PO2] 160 mmHg at sea level) to the mitochondria in tissues (PO2 3–20 mmHg). The gaseous waste product of aerobic metabolism, carbon dioxide (CO2), is assisted in its transport to atmospheric air like O2, but unlike O2, diffuses readily across tissues.
The fraction of inspired O2 (Fio2) in atmospheric air is 21%, but the partial pressure of inspired O2 (PIO2) is also dependent on barometric pressure (PB), primarily as a function of altitude. O2 entering the alveolus is “diluted” by humidification (PH2O = 47 mmHg), and CO2 in the alveolus. Arterial partial pressure of CO2 (PaCO2) approximately equals alveolar partial pressure of CO2 (PAco2) due to ready diffusion across capillary and alveolar membranes. PACO2 is a function of energy metabolism (production of CO2) and minute ventilatory volume (elimination of CO2). CO2 production reflects the respiratory quotient (RQ); RQ of a balanced diet is ~0.83. CO2 elimination is reflected by the PACO2. CO2 is also involved in acid–base balance, which can impact its rate of elimination.
The simplified alveolar gas equation calculates an estimate of alveolar partial pressure of O2 (PAO2): PAO2 ~ PIO2 – (PaCO2/RQ). At sea level in atmospheric air, PB is 760 mmHg. The resulting Pio2 is Pio2 = (FIO2 *(PB–PH2O), or (0.21*(760 – 47)) = 149 mmHg. In the alveolus, CO2 is present in proportion to the RQ and minute ventilation, and the PIO2 is diminished by the factor PaCO2/RQ. With a PACO2 = paco2 of 40 mmHg and an R of 0.83, the ideal PAO2 at sea level is 149 mmHg – (40/0.83) = 101 mmHg. In contrast, in Salt Lake City, Utah, 1280 m above sea level, the PB is 647 mmHG, and the ideal PAO2 is 77 mmHG. Hyperventilation, environment, diet, and disease can influence PAO2 through their impact on FIO2, PaCO2, and RQ in different ways. For example, hyperventilation to a PaCO2 of 30 mmHg increases PAO2 from 77 to 89 mmHg in Salt Lake City. Hyperventilation refers to an increase in minute ventilatory volume by increasing tidal volume, respiratory rate, or both.
Diffusion of O2 across the alveolar and pulmonary capillary membranes takes time and is affected by diffusion distance and Pao2–pulmonary venous Po2 gradient. Uptake of O2 in pulmonary capillary blood is a function of diffusion time, transit time/amount of blood flow through the capillary bed, and O2–hemoglobin affinity. The complex air and blood flow control processes in the lungs include mechanisms to regulate matching of ventilation (V) and perfusion (Q). The partial pressure of arterial O2 (Pao2) is also influenced by deoxygenated blood that bypasses the alveolar capillary bed, referred to as the shunt fraction. Together, these result in a “normal” alveolar-arterial (A-a) oxygen gradient, or difference between Pao2 and Pao2. The anatomic, or fixed, shunt fraction is about 2% of cardiac output and combines with a variable physiologic shunt fraction in part reflecting imperfect ventilation-perfusion (V/Q) matching to create the A-a gradient. Accordingly, the normal (measured) Pao2 of blood at sea level is ~95 mmHg and in Salt Lake City, ~72 mmHg. A Pao2 of 60 mmHg is thought to trigger physiologic mechanisms to acclimate to chronic hypoxemia.2 Diseases altering diffusion, lung ventilation, pulmonary perfusion, V/Q matching, and fixed shunt fraction can impact Pao2.
Once O2 crosses the alveolar-pulmonary capillary membranes, a minimal amount is dissolved in plasma (0.003 mL per dL of blood for each mmHg of Pao2). The majority is transported bound to hemoglobin reversibly as oxyhemoglobin. When 100% of the O2 binding sites are occupied, hemoglobin carries 1.34 mL (at standard temperature and pressure [STP]) of O2 per gram. The O2 binding relationship with hemoglobin tetramers is complex, generating an S-shaped curve of O2 percent saturation of hemoglobin binding sites when viewed graphically (Figure 28-1). This hemoglobin saturation–desaturation relationship facilitates O2 uptake across the alveolar-pulmonary capillary membranes and O2 release in tissues, where PO2 ranges between 3 and 20 mmHg. Several substances and conditions can “shift” the oxyhemoglobin saturation curve rightward, toward decreased pulmonary O2 uptake and improved tissue release, or leftward, leading to improved pulmonary O2 uptake and inhibited tissue release (see footnote, Figure 28-1). The capacity of blood to carry O2 (CaO2) is dependent on hemoglobin content and is a combination of dissolved O2 and that carried by hemoglobin.
FIGURE 28-1.
Oxyhemoglobin saturation-desaturation curve. The normal curve for adult hemoglobin is depicted, as are examples of left-shifted (poor tissue O2 unloading) and right-shifted (improved tissue O2 unloading) curves. The curve is shifted to the left by alkalemia, carboxyhemoglobinemia, hypocarbia, hypothermia, methemoglobinemia, high fetal hemoglobin content, and low 2,3-diphosphoglycerate (2,3-DPG). The curve is shifted to the right by acidemia, hypercarbia, hyperthermia, sulfhemoglobinemia, and high levels of 2,3-DPG, an end product of red cell metabolism. Note the Po2, at which 50% of the O2 is unloaded for each curve, and the range of tissue Po2 (thick bar from 3 to 20).

Disease processes that impact CaO2 include anemia, impeded hemoglobin O2 binding, and any disease or other condition resulting in a reduction in PAO2 or PaO2 (see above). For example, the majority of O2 carried by the blood is bound to hemoglobin, therefore anemia results in decreased CaO2. Acute isovolemic anemia is tolerated to a hemoglobin level of 5–7 g/dL or CaO2 of 7–10 mL O2/dL blood.3-5 Chronic anemia is tolerated below 5 g/dL, but the “low threshold” in chronic anemia that is incompatible with life is unknown, with levels as low as 1.5 g/dL documented.6 Cyanosis, which is clinically visible deoxyhemoglobinemia, is detected at a capillary deoxyhemoglobin concentration of 4 to 6 g/dL, or arterial hemoglobin saturation of about 80% with hemoglobin of 15 g/dL; however, cyanosis is a function of perfusion, tissue O2 extraction, and other factors apart from arterial hemoglobin O2 saturation.7-9 In a polycythemic individual, such as a newborn, the threshold for clinically visible cyanosis may occur at higher overall hemoglobin O2 saturations.
Delivering oxygen to tissue beds (DO2) is dependent on CaO2 × cardiac output (CO; a function of stroke volume and heart rate) and requires an open vascular supply. In the capillary beds, O2 delivery is dependent on oxyhemoglobin dissociation, capillary–tissue PO2 gradient, and distance from the capillary vessel to the tissue cells. Once O2 is delivered, there must be viable, functional cells/mitochondria to ensure O2 use. Tissue hypoxia results when delivery of O2 cannot meet O2 demand which may reflect impaired delivery or increased metabolic needs, or both. Generalized tissue hypoxia is reflected in the mixed venous O2 partial pressure indicating maximal O2 extraction in the capillary beds along a steep O2 partial pressure gradient. Examples of clinical disease affecting DO2 include heart failure, septic shock, peripheral arterial occlusion, and anything affecting CaO2. Common physiologic responses to impaired DO2 include tachycardia and hyperventilation to maximize Pao2.10
The need for oxygen in tissue beds drives the complex process of delivering O2 from atmospheric air, along its partial pressure gradient, to tissues. Understanding the process helps in understanding clinical physiologic responses to hypoxia (hyperventilation/tachypnea, increased cardiac output/tachycardia, polycythemia, V/Q matching, etc.) and pathophysiologic disequilibrium (V/Q mismatch, increased shunt fraction, shock, hyperventilation, respiratory failure, etc.). The most common clinical manifestations of disease resulting in a risk for tissue hypoxia result in blood hypoxemia as well, although important exceptions exist.
DEFINITIONS
Hypoxia is a relative or absolute deficiency of tissue O2; anoxia is the complete lack of O2. Hypoxemia is a subset of hypoxia, referring specifically to low oxygen (O2) levels in arterial blood possibly associated with secondary tissue hypoxia. There are four broad categories of O2 deprivation that may lead to tissue hypoxia:11
-
Hypoxemic hypoxia describes low blood O2 levels from pulmonary or environmental causes (e.g. pneumonia with V/Q mismatch, high altitude, interstitial lung disease, shunting from congenital heart disease).
-
Hypemic hypoxia describes decreased blood O2-carrying capacity (e.g. anemia, carbon monoxide poisoning, methemoglobinemia).
-
Ischemic or stagnant hypoxia describes decreased tissue O2 delivery (e.g. shock, arterial occlusive disease).
-
Histotoxic hypoxia describes mitochondrial cytochrome poisoning (e.g. cyanide, carbon monoxide).
PATIENT HISTORY
The history related to hypoxemia includes a complete pulmonary systems review, including infectious symptoms; this covers most clinical presentations. A history of possible toxic exposure or inhalation can help diagnose some conditions not easily detected by pulse oximetry (e.g. carbon monoxide poisoning; see Chapter 184). Review of the history with regard to underlying lung disease, cardiovascular disease, medication use, prior thoracic surgery, chest wall deformity or restriction (e.g. scoliosis), allergy, prior or current O2 baseline use, prior need for artificial ventilatory support, and results of prior blood gas testing, pulmonary function tests, or other studies of cardiopulmonary function are also essential. Generalized symptoms of hypoxemia, such as poor feeding, altered mentation, and decreased level of activity should be sought.
The physical examination should include a critical evaluation of the patient’s vital signs (including pulse oximetry, SpO2) based on norms for age and altitude (Table 28-1), since tachycardia and tachypnea can compensate for relatively mild hypoxemia resulting in a normal SpO2 reading. An initial impression of the patient’s degree of illness is beneficial, because hypoxia (with or without hypoxemia) may be present if the patient looks ill or uncomfortable. A careful cardiopulmonary examination should include a search for peripheral evidence of hypoperfusion or regional or systemic hypoxia and for chest wall abnormalities. A neurologic evaluation with regard to respiratory drive, strength of the muscles of respiration, and level of consciousness (as the brain is the most sensitive tissue to hypoxia) is applicable. If the patient has been placed on O2, the degree of impairment may be masked, leaving a false impression of less acute illness. A review of findings prior to initiating O2 therapy with persons who observed or examined the patient can be helpful. It should also be noted that significant deoxyhemoglobinemia cannot be easily achieved in an anemic patient, so cyanosis is not a reliable physical finding of hypoxemia with coexistent anemia. A number of studies have undertaken the objective of identifying clinical findings that will predict hypoxemia. No history or physical examination findings, or combination of findings, have been shown to have sufficient sensitivity and specificity to reasonably predict hypoxemia; however, findings that consistently appear as better predictors include cyanosis (lower sensitivity, high specificity), increased work of breathing (retractions, grunting, head bobbing, nasal flaring), tachypnea >50–70 depending on age, poor feeding, and altered mentation (lethargy, decreased alertness, sleepy) (the latter 4 with high sensitivity, lower specificity).12,13
0 – 1000M | 1500 – 2500M | 3600-4100M | Published* | |||||||||
Age | HR | RR | SpO2% | HR | RR | SpO2% | HR | RR | SpO2% | HR | RR | Age |
Birth | 90-163 | 26-68 | 89-100 | 88-97 | 85-95 | 70-190 | 24-50 | NB | ||||
1 mo | 108-182 | 25-67 | 87-100 | 87-98 | ||||||||
3 mo | 105-179 | 24-66 | 97-100 | 86-97 | 71-81 | |||||||
1-5 mo | 104-177 | 23-67 | 87-100 | 123-155 | 36-62 | |||||||
6-11 mo | 93-172 | 22-63 | 95-100 | 117-149 | 31-53 | 80-160 | 24-38 | 1-11 mo | ||||
12-23 mo | 80-159 | 17-57 | 96-100 | 96-100 | 108-138 | 25-41 | 85-90 | 80-130 | 22-30 | 2 y | ||
24-35 mo | 73-148 | 16-43 | 96-100 | 104-140 | 26-38 | 80-120 | 20-24 | 4 y | ||||
36-60 mo | 64-139 | 16-35 | 96-100 | 91-123 | 22-35 | 75-115 | 20-24 | 6 y | ||||
2-6 y | 62-147 | 16-43 | 96-100 | 70-110 | 18-24 | 8 y | ||||||
7-11 y | 52-123 | 13-26 | 99-100 | 70-110 | 16-22 | 10 y | ||||||
12-16 y | 45-116 | 10-24 | 96-100 | 55-105 | 14-20 | 14-18 y |
Pulse oximetry combines the principles of spectrophotometry and optical plethysmography, taking advantage of the differential absorption of light in the red and ultraviolet wavelengths of deoxy- and oxyhemoglobin, and differentiating arterial flow as pulsatile flow14. The probe includes two light-emitting diodes and a photo detector placed opposite across a perfused tissue bed. The resulting reading is converted using an empirically determined algorithm and reported as the Spo2 percentage. It should be noted that each light source must transmit light across an equivalent perfused tissue bed, displayed values are delayed 3 to 6 seconds, artifacts are common, and several factors can affect the validity and accuracy of pulse oximetry (Table 28-2). Despite its limitations, pulse oximetry clearly exceeds the ability to detect hypoxemia by clinical examination.
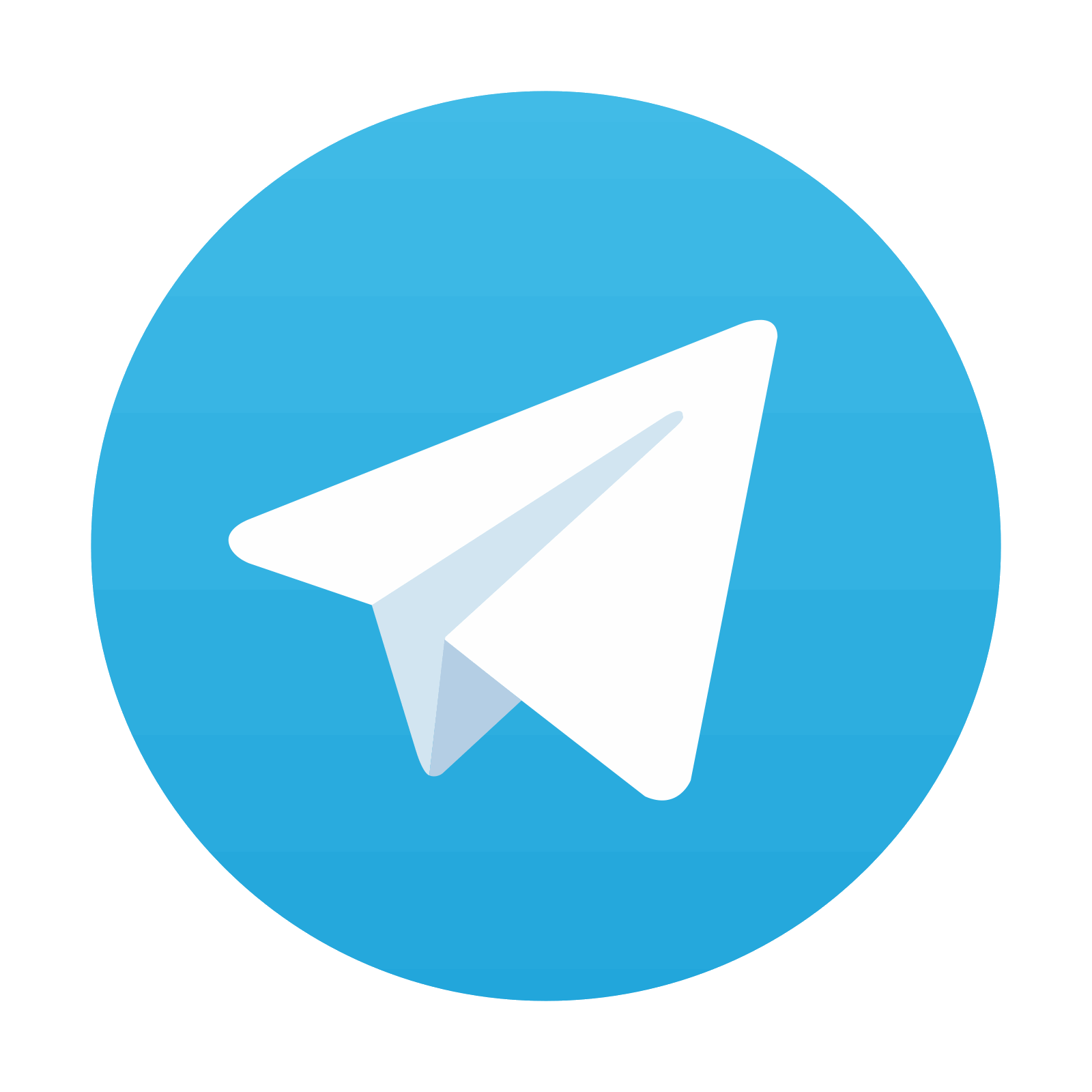
Stay updated, free articles. Join our Telegram channel
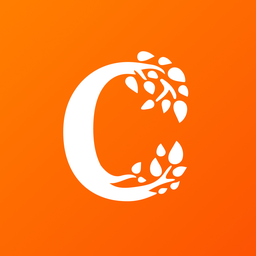
Full access? Get Clinical Tree
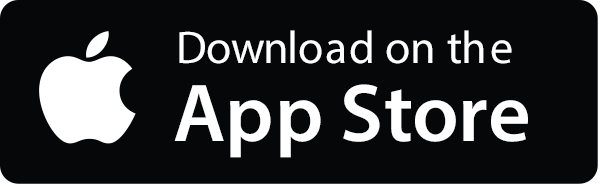
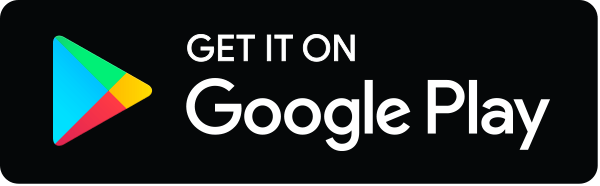