(4)
Department of Pediatrics, Service of Neonatology and Pediatric Intensive Care, University Hospital of Geneva, CH-1211, Geneva 14, Switzerland
45.1.1 Indication and General Considerations
The classical approach was to consider to switch the patient to HFOV when conventional modes of ventilation fail to provide adequate oxygenation or adequate alveolar ventilation (i.e. rescue HFOV) in infants with respiratory distress syndrome given the more recent understanding that “non-lung-protective” mechanical ventilation, large tidal volume ventilation and/or high peak pressure ventilation or probably more high peak to PEEP pressure swings can cause or worsen lung injury in the experimental setting within a short time period (Bjorklund et al. 1997; Dreyfuss et al. 1985; Ito et al. 1997; Tremblay et al. 1997). Therefore, to initiate any lung-protective ventilator strategy from birth and/or immediately after intubation sounds reasonable. Clinical experience and recent meta-analytic data suggests strongly that initiation of HFOV relatively early in the course of evolving lung disease (Cools et al. 2010; Henderson-Smart et al. 2007) would lead to favourable outcome. A relative risk reduction (RRR) for development of chronic lung disease at 36–37 weeks postmenstrual age of 10 % in favour of elective HFOV (absolute risk reduction (ARR) of 4 %, number needed to treat (NNT) 25) has been demonstrated in a recent meta-analytic review (Henderson-Smart et al. 2007). Furthermore, using HFVO from intubation together with deliberate lung volume recruitment manoeuvres has been reported in a observational study to have excellent outcome (Rimensberger et al. 2000).
Classical indications for HFOV in the neonatal population are infant respiratory distress syndrome; congenital diaphragmatic hernia, usually associated with alveolar and pulmonary vascular hypoplasia (Nobuhara et al. 1996); air-leak syndrome such as pneumothoraces or interstitial emphysema; and persistent pulmonary hypertension of the newborn (PPHN).
45.1.2 Initial Settings on HFOV
When initiating HFOV directly from intubation (“first intention approach” (Rimensberger et al. 2000)), mean airway pressures (P AW) have to be chosen low (i.e. 6–8 cm H2O) and followed according to the underlying pathology by a slow stepwise increase in CDP when oxygen requirements are high (FiO2 > 0.4). When transitioning the patient to HFOV from CMV, the P AW is typically set 3–4 cm H2O above the mean airway pressure last used on the conventional ventilator to match previous oxygenation. This can be explained by the fact that during HFOV, because of the small pressure swings, the recruitment effect of the tidal breath (or of the positive inspiratory pressure) on CMV (Richard et al. 2001) will get partly lost resulting in hypoxaemia (Fig. 45.1).
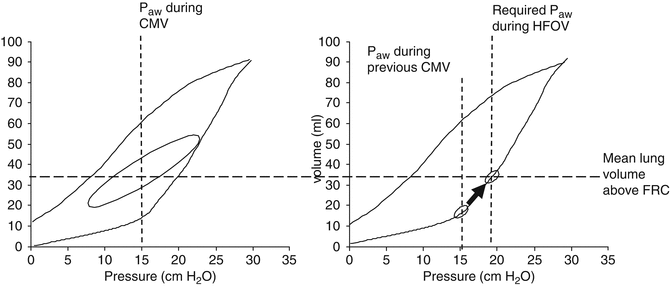
Fig. 45.1
To restore loss in mean lung volume and therefore oxygenation when switching from CMV to HFOV, the mean Paw has to be increased by 3–4 cm H2O
Amplitude is initially set by adjusting the pressure swings amplitude to observe visible chest wall vibrations, although it is difficult to define how much is visible enough. A simple method at bedside, at least in the premature infant, is to use two fingers, one positioned on the upper left and the other simultaneously on the upper right chest, and feel the intensity and symmetry of vibrations. This method allows to detect easily one-sided intubation and can become, for an experienced physician or nurse, a simple tool for titration of initial amplitude settings. The adequacy of the chosen pressure amplitude has to be controlled rapidly by a pCO2 measurement (eventually by transcutaneous measurements) especially in the preterm infant to avoid as possible any hypocarbia that is undesirable because of increasing the risk of cerebral adverse outcome (Giannakopoulou et al. 2004; Blumenthal 2004). Clinically and in absence of heavy sedation that is usually not needed in neonates while on high-frequency oscillation, the observation that the baby has spontaneous breathing efforts is reassuring because it is unlikely, in absence of major metabolic acidosis, that the baby is over-ventilated at this moment. In fact, neonates can breathe spontaneously during HFOV, the relative high bias flow of the various available neonatal HFOV machines allowing for it (van Heerde et al. 2006).
In actual clinical application, initial frequencies are mainly chosen according the age of the patient and the clinical pathology (Fig. 45.2). Although such an approach is pragmatic and would be easy to use at bedside, it cannot always be fully recommended. Because of the concept of corner frequency of the lung, low-compliance lungs are optimally ventilated at rather high frequencies, while lower frequencies are more appropriate in high-resistance systems (see Sect. 8.2.1.1.1.2) (Pillow 2005; Venegas and Fredberg 1994). In such, using the maximum amplitude coupled with the highest frequency affordable to achieve acceptable CO2 values independent of age is, in restrictive lung disease (i.e. lungs with poor compliance), the best approach (Bryan and Cox 1999; Fessler et al. 2007).
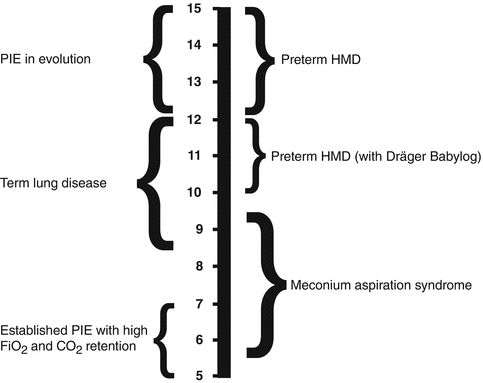
Fig. 45.2
Recommended initial frequency ranges for various neonatal pathologies (Courtesy from Peter Dargaville, Hobart, Australia)
Therefore, since most of neonatal lung pathologies are characterised by low-compliance lungs associated to or not increased airway resistance, it is hardly necessary to lower frequencies below 10 Hz in neonates.
45.1.3 Adjusting Ventilator Settings During HFOV
During HFOV oxygenation and ventilation can be under normal conditions separated, the mean airway pressures (P AW) being directly related to lung volumes and therefore oxygenation, as long as there is reversible intrapulmonary shunt. Amplitude and frequency changes, in order of priority, have to be used for modifying ventilation efficiency (i.e. CO2 washout). Alveolar minute ventilation is a function of rate (in Hz) and the squared tidal volume (in ml) (
). However, the two parameters are not independent from each other. Frequency controls the time allowed (distance) for the piston or membrane to move. Therefore, the lower the frequency, the greater the volume displaced, and the higher the frequency, the smaller the volume displaced (Fig. 45.3). In consequence, to increase CO2 washout the amplitude has to be increased, and if this is insufficient, the frequency has to be lowered.
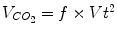

Fig. 45.3
Frequency controls the time allowed (distance) for the piston to move. Therefore, the lower the frequency, the greater the volume (indicated by the area under the curve) displaced, and the higher the frequency, the smaller the volume displaced
45.1.4 Respiratory Monitoring During HFOV
Because of the oscillatory pressures, swings accelerated molecular diffusion along the airways with a very efficient CO2 washout during HFOV, and continuous pCO2 monitoring becomes an obligation during HFOV, especially in the premature infant. With the disappearance of intravascular fibre optic small catheters that allowed for continuous blood-gas measurements, the only method that still can offer such continuous monitoring is transcutaneous pCO2 monitoring. Despite important technical evolution of this method recently, a certain offset between real PaCO2 and TCO2 is often observed (depending form skin perfusion and heating capacity of the sensor). Although this might be seen as a major inconvenience, it does not question the usefulness of continuous tcPCO2 measurements at bedside, since the offset remains stable over several hours as long as the measuring site or the sensor temperature is not changed. Therefore, trend monitoring is feasible over time and important, since every change in amplitude and/or CDP may induce a rapid change in pCO2 values (Fig. 45.4) that would be missed by doing a blood-gas measurement only 20–30 min after a change of a ventilator setting, as classically recommended in clinical practice. End-tidal CO2 measurements cannot be used during HFOV.
45.1.5 The Use of HFOV in Various Pulmonary Pathologies of the Newborn
45.1.5.1 Infant Respiratory Distress Syndrome (iRDS)
For improving oxygenation in a recruitable lung, iRDS being the optimal example for a highly recruitable lung pathology, at least in the early stage of iRDS before widespread formation of hyaline membranes, cellular infiltrations and fibrinous depositions occur, and if applying an “open-lung” strategy, mean airway pressures (P AW) are gradually increased by steps of 1–2 cm H2O with the goal of reducing the FiO2 to ≤ 0.4 while achieving adequate SaO2 values (≥90 %, and ≥85 % in the premature infant). During this phase, CO2 and haemodynamics should be carefully monitored, and at the occurrence of a minimal increase of CO2 (indicating beginning overdistention) or a decrease of blood pressures (i.e. more than 2 mmHg in the preterm infant), airway pressures have to be reduced despite eventual still poor oxygenation and high FiO2 needs, before eventually further increasing airway pressures again. Once appropriate saturation is achieved and FiO2 could be lowered to ≤0.4 (e.g. in experienced hands ≤0.3), airway pressure should be reduced stepwise in increments of 1–2 cm H2O (moving downward on the deflation limb of the PV-curve that exhibits hysteresis (Bryan and Cox 1999; De Jaegere et al. 2006; Pellicano et al. 2009)) as long as SaO2 can be maintained, the ultimate goal being to find the lowest pressure needed to maintain “good” saturation (Fig. 45.4). Chest radiography is usually recommended after finding the “optimal” mean airway pressures (P AW) to ensure adequacy of lung recruitment and to exclude signs of overinflation, although only gross overinflation can be seen on chest X-ray by the loss of the dome shape of the diaphragm. If signs of overinflation are present, airway pressures have to be reduced further. The common definition of a good lung volume by the position of both hemidiaphragms at the level of the 8th to 9th rib lacks any scientific evidence.
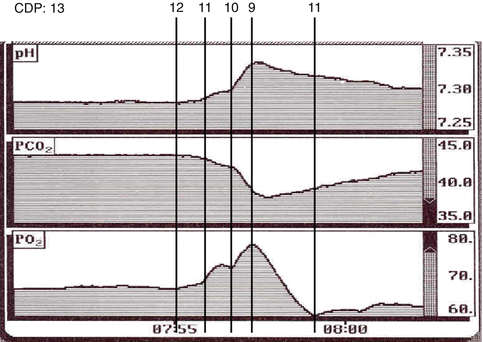
Fig. 45.4
Continuous intra-arterial blood-gas monitoring from a VLBW infant with a “stable” respiratory condition according blood-gas readings. A stepwise reduction of the CDP from initially 13–10 cm H2O (without any frequency or amplitude change on the high-frequency oscillator, Sensormedics A) leads to a rapid (!) drop in PaCO2 and an increase in PaO2, suggesting that 13 cm H2O of CDP had led to significant lung overdistention. When reducing CDP one step further from 10 to 9, a sudden drop of paO2 and a rapid increase in PaCO2 can be observed, indicating that progressive lung closing starts to happen at this pressure level (i.e. closing pressure). Reincreasing the CDP by 2 cm H2O to 11 cm H2O seems not to be enough to re-recruit the lung sufficiently again. After re-recruitment the “optimal CDP” (i.e. lowest pressure that allowed to prevent lung collapse) for this patient was 10 cm H2O
45.1.5.2 Meconium Aspiration Syndrome (MAS)
Meconium aspiration syndrome is characterised by a variable combination of atelectasis, airflow obstruction and lung inflammation, and is often accompanied by pulmonary hypertension. Maldistribution of ventilation and aeration is therefore common (Yeh 2010), rendering usually efficient but lung-protective ventilation difficult with conventional ventilation. Interestingly, HFOV (see Sect. 47.2.3 for indications) allows often to achieve relative good pulmonary ventilation and gas exchange (Clark et al. 1994) reducing the need for extracorporeal membrane oxygenation (ECMO) treatment and decreasing the incidence of air leaks.
With poor oxygenation due to an important intrapulmonary shunt (predominant atelectasis), stepwise lung recruitment attempts as described above are justified and relatively high mean airway pressures (P AW) might be required, whereas with poor oxygenation because of an extrapulmonary right to left shunt (with suprasystemic pulmonary artery pressures due to acidosis – acidosis being an important pulmonary vasoconstrictor) and/or lung overdistention, high mean airway pressures or formal recruitment attempts are often inefficient and may be in fact dangerous. This is because of the risk of further overdistending the lungs and often poor haemodynamic tolerance (drop in systemic blood pressure and often oxygenation, as well as the risk of exacerbating pulmonary hypertension). In any case, after any recruitment attempt, whether beneficial or not in terms of oxygenation and ventilation efficiency, airway pressures have to be immediately lowered to the lowest pressure needed to maintain adequate lung volumes. Because of the high probability of having to deal with high airway resistance, low frequencies below 10 Hz can in general be advocated (Hachey et al. 1998). For more detailed informations on MAS, see Sect. 47.2.3.
45.1.5.3 Lung Hypoplasia
Lung hypoplasia syndromes, of which congenital diaphragmatic hernia (CDH) is part of (see Sect. 47.2.4), are characterised by a reduced total lung capacity in relation to body weight. This means that using “only” 4–5 ml/kg of tidal volume (Vt) during conventional ventilation might be inappropriately high and is likely to cause secondary lung injury. However, using lower Vt than 3–4 ml/kg will render ventilation in terms of CO2 washout difficult and not be clinically acceptable in a situation when important respiratory acidosis (i.e. permissive hypercapnia) might haemodynamically not well be tolerated because of already coexisting pulmonary hypertension. To switch to HFOV as an early intervention or even first intention approach as soon as PIP exceeds 25 cm H2O during conventional ventilation has therefore to be proposed. However, to use a high lung volume approach as commonly recommended during HFOV would be inappropriate since lung hypoplasia syndrome does, like CDH, not represent per se a recruitable lung. The peak-to-peak pressures (amplitude) are adjusted to achieve a PaCO2 in the range of 35–45 mmHg with a pH in the range of 7.35–7.45 as long as the preductal SaO2 is >85 %. Mean airway pressure is limited in absence of any additional restrictive lung disease (e.g. iRDS) to 14–16 cm H2O.
45.1.5.4 Air-Leak Syndromes
HFOV was established years ago as the choice of a ventilator mode (i.e. rescue treatment) for pre-existing pulmonary air-leak syndromes (PAL) resulting from injurious mechanical ventilation. This was mainly based on a single uncontrolled rescue study (Clark et al. 1986). Furthermore, the use of HFOV was shown to reduce the risk [RR 0.73 (0.55, 0.96), RD −0.174 (−0.321, −0.027), number of infants that need to be treated (NNT) 6 (95 % CI 3, 37)] of developing any new pulmonary air leak (interstitial pulmonary emphysema and/or pneumothorax) in infants ventilated for severe RDS (Bhuta et al. 2001). However, this latter analysis is based on the results of a single randomised controlled trial (HiFO Study Group 1993), and no benefit of HFOV was shown for those infants with established moderate to severe PIE at entry into the trial.
While HFOV might be accepted as a suitable rescue ventilation mode for PAL, the selection of oscillatory frequency is an unresolved issue: selecting the wrong frequency can potentially exacerbate the underlying problem of air leak. In an optimal setting, frequency would be determined by measuring the impedance of the lung and determination of the corner frequency, which defines the optimal frequency in the overdamped newborn lung (Pillow 2005; Venegas and Fredberg 1994). However, as this approach is currently only used in a research setting, clinicians have little to guide them on optimal frequency selection. In general, increased resistance will decrease the corner frequency, whereas decreased compliance will increase the corner frequency. Corner frequency is also dependent on patient size and is higher in smaller infants. Whereas some advocate using a low HFOV frequency for air leak, there are two major concerns with this approach. Firstly, by using a fixed % setting of inspiratory time, the inspiratory time will increase as frequency decreases – so the duration of exposure to the high pressure is longer and higher tidal volumes will be delivered. Secondly, lower resistance and inertance at lower frequency results in a higher percentage of the airway-opening pressure amplitude being delivered to the proximal and distal airspaces (see Sect. 5.2.1.1). Although the higher respiratory resistance in PAL potentially justifies a lower frequency compared with a poorly compliant lung (i.e. classical iRDS), decreasing frequency below 8–10 Hz is probably not justified based on respiratory mechanics (except in very big infants). Excessively low frequencies potentially expose the central airways to large-pressure oscillations as well as those airspaces proximal to areas of high peripheral resistance. Decreasing the % inspiratory time to 30 % or below to limit delivered tidal volume would reduce volutrauma associated with the use of lower frequencies.
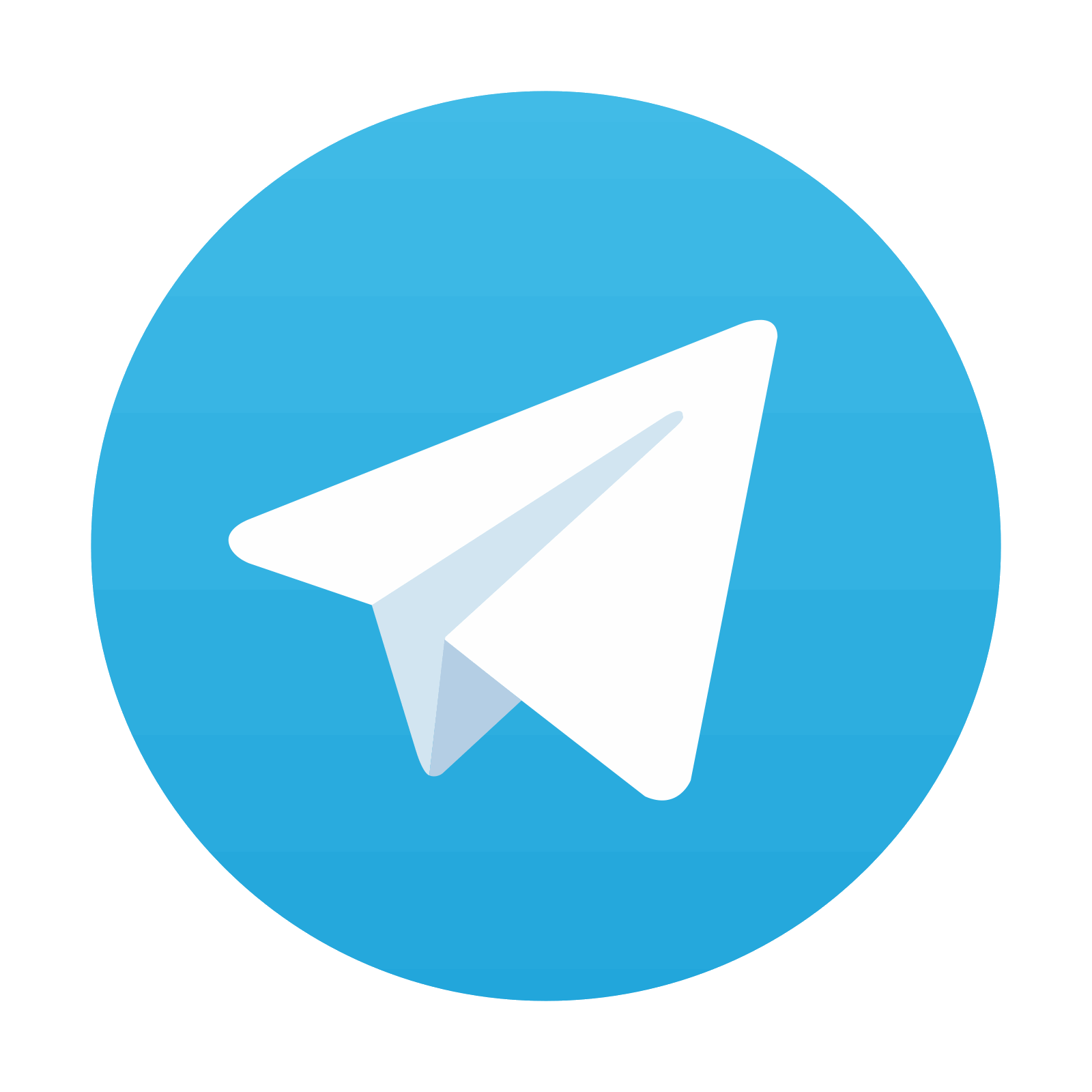
Stay updated, free articles. Join our Telegram channel
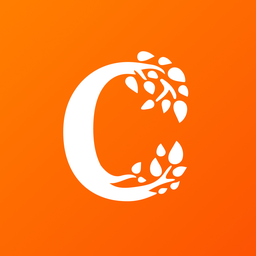
Full access? Get Clinical Tree
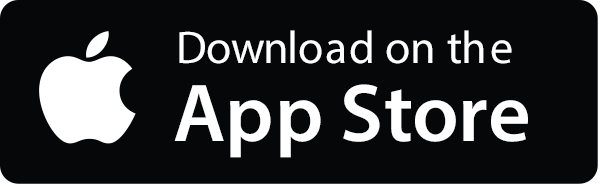
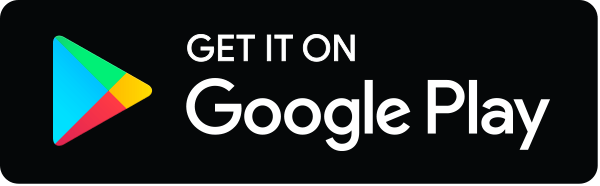