-
Chapter Contents
-
Developmental haemopoiesis 756
-
Neonatal anaemia and other red cell disorders 758
-
Introduction and definition 758
-
Physiological impact of anaemia in the neonate 759
-
Pathogenesis and causes of neonatal anaemia 760
-
Neonatal anaemia due to reduced red cell production 760
-
Anaemia due to increased red cell destruction (haemolytic anaemia) 761
-
Anaemia due to blood loss 765
-
Anaemia of prematurity 766
-
A simple diagnostic approach to neonatal anaemia 767
-
Polycythaemia 767
-
-
White cell disorders 768
-
Haemostasis and thrombosis in the newborn 770
-
Introduction 770
-
Developmental haemostasis 772
-
Diagnostic approach to bleeding in neonates 772
-
Inherited coagulation disorders 773
-
Acquired disorders of coagulation 775
-
Normal thrombocytopenia 777
-
Neonatal thrombosis: physiology and developmental aspects 778
-
Diagnostic approach to neonatal thrombosis: use of screening tests 778
-
Inherited thrombotic disorders 779
-
Acquired thrombotic problems 780
-
-
Transfusion of blood and blood products in the newborn 780
-
Introduction 780
-
Red cell transfusion 781
-
Aims of red cell transfusion 781
-
Changing patterns of red cell transfusion in neonates 781
-
Guidelines for transfusion of red cells in neonates 782
-
Products for red cell transfusion in neonates 782
-
Indications for red blood cell transfusion in preterm neonates 782
-
Red blood cell T-antigen activation 782
-
-
Platelet transfusion 782
-
Granulocytes 783
-
Fresh frozen plasma and cryoprecipitate 783
-
Human albumin solution 784
-
Exchange transfusion 784
-
Developmental haemopoiesis
Introduction
The process which ensures lifelong production of all haemopoietic cells is known as haemopoiesis. Sequential changes in the regulation of haemopoiesis during development help to explain the natural history of many neonatal haematological problems. Haemopoiesis in humans begins in the yolk sac in the third week of gestation ( ). By 5 weeks’ gestation, the main site of definitive haemopoiesis is found in the aorto-gonad-mesonephros region of the dorsal aorta. Haemopoiesis in the aorta is only transient and haemopoietic stem cells migrate from there a few weeks later to the liver, which remains the main site of blood cell production throughout fetal life ( ; ). Even though signs of haemopoiesis are also found in the bone marrow from 11 weeks’ gestation, this makes only a small contribution to overall haemopoiesis until after birth ( ). Thus, for preterm infants, the liver is the main haemopoietic organ at and shortly after birth; this is likely to be a contributory factor in a number of disorders, including the haematological abnormalities seen in neonates with Down syndrome (see later sections).
Erythropoiesis in the fetus and neonate
Erythropoiesis, production of red blood cells (RBCs), has a number of distinct characteristics in term and preterm neonates compared with older children which are relevant to our understanding of neonatal anaemias:
- •
Rate of haemoglobin synthesis and RBC production : the rates of haemoglobin synthesis and of RBC production fall dramatically after birth and remain low for the first 2 weeks of life, probably in response to the sudden increase in tissue oxygenation at birth ( ). The physiological rise in RBC production begins several weeks later and by 3 months of age a healthy infant, whatever the gestation at birth, should be able to produce up to 2 ml of packed RBCs per day ( ). Studies in preterm neonates suggest that over the first 2 months of life the maximal rate of RBC production is about 1 ml/day since preterm babies receiving erythropoietin are unable to maintain their haemoglobin if >1 ml of blood per day is venesected for diagnostic purposes but can do so when sampling losses are less than this ( ).
- •
Reduced RBC lifespan : neonatal RBCs, particularly from preterm babies, have a reduced lifespan compared with adult red cells. Calculated RBC lifespans for preterm infants are 35–50 days compared with 60–70 days for term infants and 120 days for healthy adults ( ). The main reason for this is the many differences in the membrane of neonatal versus adult RBCs, including increased resistance to osmotic lysis, increased mechanical fragility, increased total lipid content and an altered lipid profile, increased insulin-binding sites and reduced expression of blood group antigens such as A, B and I ( ).
- •
Altered RBC metabolism : there are numerous differences in the glycolytic and pentose phosphate pathways between neonatal and adult RBCs which lead to an increased susceptibility to oxidant-induced injury ( ). In addition, neonatal RBCs have reduced levels of NADH methaemoglobin reductase (about 60% of those in adult RBCs). This makes them more likely to develop methaemoglobinaemia as they are more susceptible to the toxic effects of chemicals (e.g. nitric oxide) which oxidise haemoglobin iron more rapidly than the maximal rate of methaemoglobin reduction; methaemoglobin levels are correspondingly slightly higher in neonates than in adults (mean 0.43 g/dl in preterm neonates, 0.22 g/dl in term neonates and 0.11 g/dl in adults) ( ).
- •
Changes in globin chain synthesis in the fetus and newborn : the first globin chain produced is epsilon globin, followed almost immediately by α- and γ-globin chain production ( Table 30.1 ). HbF (α 2 γ 2 ) is therefore produced from early in gestation (4–5 weeks) and is the predominant haemoglobin until after birth. Adult haemoglobin (HbA: α 2 β 2 ) remains at low levels (10–15%) until 30–32 weeks. After this, the rate of HbA production increases at the same time as HbF production falls, resulting in an average HbF level at term birth of 70–80%, HbA of 25–30%, small amounts of HbA 2 and sometimes a trace of Hb Barts (β 4 ) ( ). After birth, HbF falls, to ~2% at age 12 months with a corresponding increase in HbA. In term babies there is little change in HbF in the first 15 days after birth. In preterm babies who are not transfused, HbF may remain at the same level for the first 6 weeks of life before HbA production starts to increase. It is this delay in HbA production (i.e. the switch from the γ-globin of HbF to the β-globin of HbA) which can make the diagnosis of β-globin disorders difficult in the neonatal period. By contrast, the fact that α-globin chains are absolutely essential for the production of both HbF and HbA means that α-thalassaemia major causes severe anaemia from early in fetal life ( ).
Table 30.1Composition of haemoglobins in the human embryo, fetus and neonateHAEMOGLOBIN GLOBIN CHAINS GESTATION α-GLOBIN GENE CLUSTER β-GLOBIN GENE CLUSTER * Embryonic Hb Gower-1 ζ 2 ϵ 2 From 3–4 weeks Hb Gower-2 α 2 ϵ 2 Hb Portland ζ 2 γ 2 From 4 weeks Fetal HbF α 2 γ 2 From 4 weeks Adult HbA α 2 β 2 From 6–8 weeks HbA 2 α 2 δ 2 From 30 weeks * The α-globin gene cluster is situated on chromosome 16 and the β-globin gene cluster on chromosome 11. Note that fetuses and neonates with α-thalassaemia major, who are unable to synthesise α-globin chains, will have Hb Portland as well as Hb Barts (β 4 ) detectable by haemoglobin electrophoresis or high-performance liquid chromatography.
- •
Erythropoietin production in the fetus and newborn : erythropoietin is the principal cytokine regulating erythropoiesis in the fetus and newborn ( ; ). Since erythropoietin does not cross the placenta, erythropoietin-mediated regulation of fetal erythropoiesis is predominantly under fetal control ( ). The liver is the main site of erythropoietin production in the fetus ( ) and the only stimulus to erythropoietin production under physiological conditions is hypoxia with or without anaemia. This explains the high erythropoietin levels in fetuses of mothers with diabetes or hypertension or those with intrauterine growth restriction (IUGR) or cyanotic congenital heart disease; erythropoietin is also increased in fetal anaemia of any cause, including haemolytic disease of the newborn (HDN) ( ).
White cell production in the fetus and newborn
Neutrophils
There are few circulating neutrophils in first- and second-trimester blood (0.1–0.2 × 10 9 /l) ( ), after which the numbers gradually rise to reach over 2 × 10 9 /l by term, with slightly lower numbers in preterm neonates ( Table 30.2 ). The principal difference between neutrophil production in the newborn and the adult is the reduced neutrophil storage pool, particularly in preterm infants and most markedly in IUGR or exposure to maternal hypertension ( ; ; ). The neutrophil storage pool reflects the available reserve of neutrophils which the fetus or neonate can mobilise in response to infection. This may contribute to the frequency of bacterial infection in preterm infants. The neutrophil storage pool is defined as the numbers of segmented neutrophils, band neutrophils and metamyelocytes in the bone marrow and so is usually inferred (by the leukocyte response to bacterial sepsis) since bone marrow examination is rarely indicated in the newborn. Various cytokines stimulate neutrophil production in vitro and in vivo (e.g. granulocyte colony-stimulating factor (G-CSF) and granulocyte–macrophage colony-stimulating factor (GM-CSF)) ( ; ).
BIRTH | 2 WEEKS | 2 MONTHS | |
---|---|---|---|
Hb (g/dl) | 14.9–23.7 | 13.4–19.8 | 9.4–13 |
Haematocrit | 0.47–75 | 0.41–0.65 | 0.28–0.42 |
MCV (fl) | 100–125 | 88–110 | 77–98 |
Reticulocytes (×10 9 /l) | 110–450 | 10–85 | 35–200 |
WBCs (×10 9 /l) | 10–26 | 6–21 | 5–15 |
Neutrophils (×10 9 /l) | 2.7–14.4 | 1.5–5.4 | 0.7–4.8 |
Monocytes (×10 9 /l) | 0–1.9 | 0.1–1.7 | 0.4–1.2 |
Lymphocytes (×10 9 /l) | 2.0–7.3 | 2.8–9.1 | 3.3–10.3 |
Eosinophils (×10 9 /l) | 0–0.85 | 0–0.85 | 0.05–0.9 |
Basophils (×10 9 /l) | 0–0.1 | 0–0.1 | 0.02–0.13 |
Nucleated RBCs (×10 9 /l) | <5 | <0.1 | <0.1 |
Platelets (x10 9 /l) | 150–450 | 150–450 | 150–450 |
* These data are obtained from a number of sources and have been chosen to represent data most useful for interpreting the significance of haematological results.
Monocytes, eosinophils and lymphocytes
All types of leukocyte found in adult blood are also seen in the fetus and the newborn ( ): monocytes circulate from 4–5 weeks’ gestation and eosinophils from 14–16 weeks’ gestation, increasing slowly to normal values at term (see Table 30.2 ). There are few studies of lymphopoiesis in the human fetus; both T lymphocytes and B lymphocytes are found in low numbers in fetal liver at 7 and 8 weeks’ gestation, respectively ( ), and T lymphocytes are detectable in fetal blood, marrow and thymus during the second trimester ( ). By term, T lymphocytes form 40–45% of circulating mononuclear cells, with a CD4:CD8 ratio of around 5 : 1, slightly higher than in adult blood (3.1 : 1). B lymphocytes are found in fetal blood and bone marrow from 12 weeks’ gestation and constitute 4–5% of circulating mononuclear cells by term ( ).
Megakaryocytopoiesis and platelet production in the fetus and newborn
Platelets appear in the circulation at 5–6 weeks’ gestation ( ). During the second trimester, the platelet count rises to normal adult values (175–250 × 10 9 /l) ( ). Thus, a platelet count of less than 150 × 10 9 /l is abnormal even in the most preterm neonate. The principal cytokine regulating platelet production in the fetus and newborn is thrombopoietin, as in adults ( ). There are several differences between megakaryocytopoiesis and its regulation in the fetus and newborn compared with adults which may contribute to the frequent occurrence of thrombocytopenia in sick neonates. Fetal megakaryocytes are smaller and more immature ( ; ); the numbers of megakaryocyte progenitor cells, the precursor cells for maintaining megakaryocyte and platelet production, are reduced; and the ability to produce thrombopoietin is reduced, limiting the capacity to upregulate platelet production at times of increased demand, e.g. during thrombocytopenia in neonatal sepsis ( ; ).
Neonatal anaemia and other red cell disorders
Introduction and definition
Anaemia is the commonest haematological abnormality in the newborn. In the majority of neonates, the causes are straightforward, since they reflect a combination of well-recognised physiological changes and iatrogenic blood letting. However, it is important to identify those neonates with pathological anaemia who require additional investigations and more tailored management. In the neonatal period, the most frequent diseases associated with anaemia are immune haemolysis and genetic red cell disorders. A logical approach and appropriate use of straightforward investigations, as outlined below, reveal the cause in most babies. Treatment options for neonatal anaemia are limited and are based mainly on sensible use of blood transfusion (see pp. 780–782 ) and prevention of anaemia.
Normal values for red blood cells and blood volume
Normal values at birth for haemoglobin, haematocrit and mean corpuscular volume (MCV) for term and preterm babies are shown in Table 30.2 and Table 30.3 , respectively, and in Appendix 1 .
24–25 WEEKS | 26–27 WEEKS | 28–29 WEEKS | 30–31 WEEKS | |
---|---|---|---|---|
Hb (g/dl) | 19.4 ± 1.5 | 19.0 ± 2.5 | 19.3 ± 1.8 | 19.1 ± 2.1 |
Haematocrit | 0.63 ± 0.04 | 0.62 ± 0.08 | 0.60 ± 0.07 | 0.60 ± 0.08 |
MCV (fl) | 135 ± 0.02 | 132 ± 14.4 | 131 ± 13.5 | 127 ± 12.7 |
Reticulocytes (×10 9 /l) | 279 ± 23 | 454 ± 15 | 347 ± 12 | 278 ± 10 |
Platelets (×10 9 /l) | 150–450 | 150–450 | 150–450 | 150–450 |
In term babies, the haemoglobin, haematocrit and red cell indices fall slowly over the first few weeks, reaching a mean haemoglobin of 13–14 g/dl at 4 weeks of age and 9.5–11 g/dl at 7–9 weeks of age, with a lower limit of normal for the MCV and mean corpuscular haemoglobin (MCH) of 77 fl and 26 pg, respectively. For preterm infants, these changes may be difficult to interpret, because of their variable clinical course and transfusion requirements. However, studies of well preterm infants carried out in the 1970s show a more rapid and steeper fall in haemoglobin, reaching a mean of 6.5–9 g/dl at 4–8 weeks’ postnatal age ( ). The reticulocyte count falls rapidly after birth as erythropoiesis is suppressed, and starts to increase in term babies at 7–8 weeks of age to reach 35–200 × 10 9 /l (1–1.8%) at 2 months of age, and in preterm babies at 6–8 weeks of age ( ; ; ). Normal blood volume at birth varies with gestational age and the timing of clamping of the cord ( ). In term infants, the average blood volume is 80 ml/kg (range 50–100 ml/kg), and in preterm infants is higher at 106 ml/kg (range 85–143 ml/kg) ( ; ). Term and preterm babies have adequate stores of iron, folic acid and vitamin B 12 at birth. However, stores of both iron and folic acid are lower in preterm infants and are depleted more quickly, leading to deficiency after 2–4 months if the recommended daily intakes are not maintained (see Ch. 16, part 3 ). In general, even term neonates with a normal haemoglobin at birth will have depleted their iron stores by the time they have doubled their birthweight ( ).
There are no useful published normal ranges for the numbers of circulating nucleated red cells. Nevertheless, a useful ‘rule of thumb’ is that values of less than 5 nucleated RBCs per 100 white cells in a term baby and <20 nucleated RBCs per 100 white blood cells in a preterm baby can be considered normal for the first 1–2 days of life. The commonest causes of increased numbers of circulating nucleated RBCs are:
- •
haemolysis (common in rhesus haemolytic disease and α-thalassaemia major but uncommon in other types of haemolysis)
- •
haemorrhage (especially fetomaternal haemorrhage)
- •
chronic tissue hypoxia in utero (IUGR, maternal hypertension, maternal diabetes)
- •
perinatal asphyxia.
Definition of anaemia
Anaemia can be defined as a haemoglobin concentration below the normal range for a population of age- and sex-matched individuals. Normal values for term and preterm infants at birth are shown in Tables 30.2 and 30.3 ; from this it can be seen that, regardless of gestation, any neonate with a haemoglobin of <14 g/dl at birth in a properly taken blood sample should be considered anaemic. Not every neonate with a haemoglobin concentration at birth of <14 g/dl needs detailed further investigation, but thought should be given to the cause ( Table 30.4 ) and appropriate investigations instituted. Where the reduced haemoglobin does not fit the clinical picture, the first step should be to repeat the sample, since in many cases the measured haemoglobin concentration is inaccurate because of the site of sampling (heelprick versus venous blood) or the way in which the sample was collected. The haemoglobin concentration in venous samples is lower than in heelprick samples collected simultaneously: in the first few hours of life this difference averages 2–4 g/dl and is greater at lower gestational ages ( ; ). The difference between the venous and capillary haemoglobin falls with increasing gestational age, and also with increasing postnatal age, such that by the fifth day of life there is almost no difference in haemoglobin concentration between a well-taken heelprick sample and a venous sample ( ).
Impaired red cell production |
|
Increased red cell destruction (haemolysis) |
|
Blood loss |
|
Anaemia of prematurity |
Impaired red cell production plus reduced red cell lifespan |
The influence of cord clamping
The other major influence on haemoglobin concentration at birth is the timing of cord clamping and the position of the baby at the time of clamping. In term babies, the placental vessels contain around 100 ml of blood at birth. It has been estimated that 25% of the placental blood is transfused within the first 15 seconds and 50% (i.e. 50 ml in a term baby) by the end of the first minute ( ). The difference in haemoglobin concentration in the baby between early and late cord clamping is around 3 g/dl ( ). Babies held below the level of the placenta continue to gain blood until the cord is clamped and have higher haemoglobin levels than those held above the level of the placenta, who may lose blood into the placenta until the cord is clamped ( ).
Physiological impact of anaemia in the neonate
The clinical significance of anaemia in the newborn depends on whether or not the baby is able to maintain adequate tissue oxygenation. This does not depend solely upon the haemoglobin concentration since tissue oxygenation is also influenced by cardiopulmonary function and by the ability of the haemoglobin to unload the oxygen it is carrying. Fortunately, the oxygen-unloading capacity of blood increases progressively from birth, reflecting the position of the haemoglobin–oxygen dissociation curve.
In neonates, the two most important factors determining the position of the haemoglobin–oxygen dissociation curve are the concentrations of HbF and of 2,3-diphosphoglycerate (2,3-DPG) within the RBCs: high HbF and low 2,3-DPG both cause the curve to shift to the left, i.e. the affinity of haemoglobin for oxygen is increased, so less oxygen is released to the tissues. This is the situation just after birth in both term and preterm babies, as both have HbF concentrations above 50% at birth. The high oxygen affinity may be more of a problem for preterm babies since the HbF levels are >90% in babies of 24–28 weeks’ gestation, although this may not be significant in practice since very preterm neonates are more likely to require transfusion over the first few weeks of life. Over the first few months of life, 2,3-DPG levels rise and HbF levels fall, so the haemoglobin–oxygen dissociation curve gradually shifts to the right, i.e. the oxygen affinity of haemoglobin falls and oxygen delivery to the tissues increases, to some extent ameliorating the effects of the falling haemoglobin over the first months of life.
Pathogenesis and causes of neonatal anaemia
Anaemia in the neonatal period has distinct physiological features compared with older children and a distinct pathogenesis. Interpretation of diagnostic investigations has to be made on a background of the developmental changes affecting the red cell membrane, red cell enzyme concentrations and the types and rate of haemoglobin production, which vary with gestational and postnatal age. Diagnostic tests are also often affected by whether the baby has been transfused. Furthermore, anaemia in the neonate may be due to pregnancy-related or pre-existing disorders in the mother, such as the presence of red cell alloantibodies or genetic disorders. It is therefore important to remember that in many cases evaluating the blood count and blood film of the parents is the quickest way of identifying the underlying diagnosis in the neonate.
The principal causes of neonatal anaemia are shown in Table 30.4 . In general, anaemia can result from one or more of the following mechanisms:
- •
inappropriately reduced red cell production
- •
increased red cell destruction/reduced red cell lifespan
- •
blood loss
- •
a combination of these mechanisms (anaemia of prematurity).
Neonatal anaemia due to reduced red cell production
Anaemia due to reduced red cell production is not common in the neonatal period. Nevertheless, it is clinically important, because several of the disorders that present in this way are associated with severe, lifelong problems. The main diagnostic clues to reduced red cell production are the combination of a low reticulocyte count (<20 × 10 9 /l) together with a negative direct antiglobulin test (DAT; Coombs’ test). The other useful diagnostic point is whether the disorder is confined to the red cell series (i.e. anaemia in the presence of a normal white cell and platelet count) or whether the blood count suggests that the white cells and/or platelets are also involved.
The most important causes are congenital infections (particularly due to parvovirus) and genetic disorders. Where failure of blood cell production is confined to the red cell series, as with Diamond–Blackfan anaemia (DBA) and most episodes of parvovirus infection, the anaemia is said to be due to red cell aplasia. Reduced red cell production may also be part of a general failure of haemopoiesis and accompanied by leukopenia and/or thrombocytopenia, as seen in congenital infection due to cytomegalovirus (CMV), in congenital leukaemias (see p. 770 and Ch. 36 ) and in congenital bone marrow failure syndromes such as Pearson syndrome (see p. 761 ).
Anaemia and congenital infection
Infections which cause anaemia due to reduced red cell production include parvovirus B19, CMV, toxoplasmosis, congenital syphilis, rubella and herpes simplex ( ; ). Identification of the causative organism is usually based on clinical suspicion prompted by well-recognised associated findings such as chorioretinitis, jaundice, pneumonitis, skin lesions, IUGR and hepatosplenomegaly, followed by specific diagnostic microbiological investigations. In infection due to CMV, toxoplasma or herpes simplex, the anaemia and reticulocytopenia are usually relatively mild ( ). In addition, the blood film often shows abnormal ‘viral’ lymphocytes, thrombocytopenia and/or neutropenia. Congenital parvovirus B19 can cause particular diagnostic difficulties and is discussed below ( ).
Parvovirus B19 and fetal/neonatal anaemia
Maternal infection with parvovirus B19 causes fetal anaemia which is severe enough to lead to intrauterine death in 9% of cases ( ; ; ). A diagnosis of fetal parvovirus B19 should be considered in every ‘unexplained’ case of fetal hydrops ( Table 30.5 ) since it has been estimated that parvovirus B19 is responsible for 15% of cases of non-immune hydrops ( ; ; ). In addition to anaemia, parvovirus infection causes marked reticulocytopenia (usually <10 × 10 9 /l) and thrombocytopenia may also occur. The diagnosis of parvovirus B19 infection is primarily based on maternal serology together with the demonstration of B19 DNA in the fetus/neonate by dot blot hybridisation or polymerase chain reaction (PCR) ( ; ). Where results are negative on blood samples but clinical suspicion of parvovirus is high, PCR for B19 should also be carried out on bone marrow ( ). Management depends on the severity of the anaemia – severe cases diagnosed in utero may be treated by intrauterine transfusion; for those that survive, the majority have no long-term sequelae. However, a small number of neonates with chronic red cell aplasia, with or without evidence of persistent B19 DNA by PCR, have been reported, and for such cases intravenous immunoglobulin (IVIG) should be given to try and eradicate persistent viral infection ( ; ).
Reduced red cell production |
|
Increased red cell destruction (haemolysis) |
|
Blood loss |
|
Failure of red cell production due to genetic disorders
Congenital or inherited disorders that usually or not infrequently present with neonatal anaemia due to reduced red cell production include DBA, congenital dyserythropoietic anaemia (CDA) and Pearson syndrome. The other inherited bone marrow failure syndromes, such as Fanconi’s anaemia, rarely present at birth.
Diamond–Blackfan anaemia
The principal cause of congenital red cell aplasia is DBA ( ), which has an incidence of 5–7 cases per million live births. There is a clear family history in 20% of cases (both autosomal dominant and autosomal recessive inheritance are reported); the remaining 80% are sporadic. Affected children nearly always present in the first year of life; most diagnoses are made around 2–3 months of age, but 25% of cases present at birth and rare cases present as mid-trimester fetal anaemia and hydrops ( ; ). The usual presentation is with anaemia in an otherwise healthy baby; 40% of infants have associated congenital anomalies, particularly craniofacial dysmorphism, IUGR, neck anomalies (Klippel–Feil syndrome) and thumb malformations (e.g. triphalangeal or bifid thumbs) ( ; ) similar to those seen in Fanconi’s anaemia. The important laboratory features are normochromic anaemia (which may be macrocytic), reticulocytopenia and absent erythroid precursors on the bone marrow aspirate. It is important to exclude parvovirus infection by appropriate serology and dot blot testing, and Fanconi’s anaemia by diepoxybutane stress testing of peripheral blood lymphocytes ( ). If these tests are negative, the only other differential diagnosis of red cell aplasia with absent red cell precursors is transient erythroblastopenia of childhood, which, in contrast to DBA, resolves within a couple of months. Although bone marrow examination is required to diagnose DBA, other useful tests include red cell adenosine deaminase levels, which are elevated in most affected patients and sometimes in parents ( ) and genetic analysis for mutations in ribosomal protein genes, which are present in ~50% of families and can be investigated in specialised laboratories ( ). In the neonatal period, the treatment of DBA is red cell transfusion. Up to 75% of children with DBA respond to steroids and can often be weaned off transfusions, but this approach is not usually tried until after the first year of life ( ). DBA is a rather unpredictable disease: some cases resolve spontaneously; others wax and wane; but more than half of affected children have lifelong transfusion or steroid dependence and may be treated by bone marrow transplantation ( ; ).
Congenital dyserythropoietic anaemia
The CDAs are a rare group of disorders characterised by failure of red cell production due to ineffective erythropoiesis. Most cases are autosomal recessive and a history of parental consanguinity is not uncommon. In contrast to DBA, the bone marrow has vastly increased numbers of erythroid precursors but they are grossly abnormal and do not properly differentiate into mature RBCs. CDA usually presents during childhood. However, a number of neonates with CDA presenting at birth or during fetal life have been reported and in severe cases the presentation may be with hydrops fetalis ( ). Most affected babies are normally grown with no dysmorphic features, have a normocytic anaemia with normal white cells and platelets but a low reticulocyte count and transfusion-dependent anaemia. CDA type 1 is due to mutations in the codanin-1 gene ( ) and CDA type II, which is the most common type of CDA, has recently been shown to be due to mutations in the SEC23B gene ( ). The prognosis of CDA is extremely variable. For transfusion-independent children with CDA, lifespan is usually normal and quality of life is good. For transfusion-dependent children, the treatment options are:
- •
splenectomy, which renders a small proportion of children transfusion-independent with a normal lifespan
- •
bone marrow transplantation, the only curative treatment, but one which carries a 10% risk of mortality or graft rejection
- •
lifelong red cell transfusion with iron chelation therapy, which carries a similar prognosis to children with β-thalassaemia major (i.e. median survival now >50 years with good treatment compliance).
Pearson syndrome
This rare disease is caused by mutations in mitochondrial DNA ( ). It often presents in neonates who are small-for-gestational-age and thrive poorly in the first few weeks of life ( ). The anaemia is normocytic and associated thrombocytopenia and neutropenia are common; abnormal leukocyte vacuolation may be seen in the peripheral blood and highly characteristic vacuolation of early erythoid cells on the marrow aspirate should prompt blood to be sent for mitochondrial DNA analysis to establish the diagnosis. The prognosis of Pearson syndrome is very poor: few children survive beyond the second year of life.
Anaemia due to increased red cell destruction (haemolytic anaemia)
After anaemia of prematurity and anaemia due to blood letting, haemolysis is the commonest cause of neonatal anaemia. Haemolysis should always be investigated even if the anaemia is mild and apparently trivial. This is because transient or mild haemolysis in the neonatal period may be the clue to an underlying problem with more serious manifestations later on in childhood (e.g. red cell enzymopathies) or to problems which might affect future siblings (e.g. alloimmune anaemia due to maternal red cell antibodies).
The principal clues which suggest a haemolytic anaemia are: increased numbers of reticulocytes and/or circulating nucleated RBCs, unconjugated hyperbilirubinaemia, a positive DAT (if immune) and characteristic changes in the morphology of the red cells on a blood film (e.g. hereditary spherocytosis). The main types of neonatal haemolytic anaemia are listed in Table 30.4 and a useful algorithm to guide investigations is shown in Figure 30.1 . It is usually straightforward to distinguish the cause, although rarer causes require specialist investigations which should be discussed with a haematologist once the basic investigations are to hand. The first step should be a DAT, which will be positive only in the presence of immune haemolytic anaemia and not in non-immune haemolysis. The main cause of immune haemolytic anaemia is HDN (see below). The main causes of non-immune haemolysis in neonates are:
- •
red cell membrane disorders
- •
red cell enzymopathies
- •
haemoglobinopathies.

A number of congenital and primary infections can also cause haemolytic anaemia in the neonatal period, including CMV, toxoplasmosis, congenital syphilis, rubella, herpes simplex and, rarely, malaria.
Immune haemolytic anaemias, including haemolytic disease of the newborn
By far the most common cause of DAT-positive haemolysis is HDN due to transplacental passage of maternal IgG alloantibodies to red cell antigens. Although modern DAT reagents are very sensitive, a negative DAT is sometimes found in ABO HDN due to anti-A or anti-B ( ). Maternal autoimmune haemolytic anaemia very occasionally causes a positive DAT in the neonate; however, both haemolysis and anaemia in the baby are extremely rare.
The principal alloantibodies that cause significant anaemia due to HDN are those against rhesus antigens (anti-D, anti-c and anti-E), anti-Kell, anti-Kidd (J k ), anti-Duffy (F y ) and antibodies of the MNS blood group system, including anti-U. Anti-D remains the most frequent alloantibody to cause significant haemolytic anaemia, affecting 1 in 1200 pregnancies ( ; ). Anti-Kell antibodies are less common but can cause severe fetal and neonatal anaemia since they inhibit erythropoiesis as well as causing haemolysis ( ). Most babies with HDN present with jaundice and/or anaemia and are born to women with known antibodies. In neonates with severe anaemia, there is often evidence of extramedullary haemopoiesis, including hepatosplenomegaly and occasionally skin lesions producing the clinical appearance of ‘blueberry muffin baby’ ( ; ).
HDN due to ABO antibodies
ABO haemolytic disease occurs only in offspring of women of blood group O and is confined to the 1% of such women who have high-titre IgG antibodies. Haemolysis due to anti-A is more common (1 in 150 births) than anti-B. In contrast to anti-Rhesus antibodies, both anti-A and anti-B usually cause hyperbilirubinaemia without significant neonatal anaemia. This is mainly because there are relatively few group A or B antigen sites on neonatal red cells, allowing the antibody-coated cells to persist for longer in the circulation ( ). As a reflection of this, the blood film in ABO haemolytic disease characteristically shows very large numbers of spherocytes with little or no increase in nucleated red cells; this contrasts to rhesus HDN, where there are few spherocytes and vast numbers of circulating nucleated red cells. The DAT in ABO HDN may be negative. Management of ABO HDN usually just requires phototherapy; however, close monitoring is essential and exchange transfusion is occasionally required, particularly in cases of ABO HDN due to anti-B antibodies, which may cause severe anaemia as well as hyperbilirubinaemia ( ). Hydrops has occasionally been described.
Management of HDN
The antenatal diagnosis and management of pregnancies affected by red cell alloimmunisation require cooperation between obstetric, paediatric and haematology teams ( ). All neonates at risk should have cord blood taken for measurement of haemoglobin, bilirubin and a DAT and should remain in hospital until hyperbilirubinaemia and/or anaemia have been properly managed. Phototherapy should be given from birth to all rhesus-alloimmunised infants with haemolysis, as the bilirubin can rise steeply after birth and this expectant approach will prevent the need for exchange transfusion in some infants. In HDN due to anti-Kell, anaemia is usually more prominent than jaundice and minimal phototherapy may be necessary despite severe anaemia ( ). Systematic reviews have found that treatment of neonates with alloimmune haemolysis with IVIG does reduce the need for exchange transfusion ( ; ). Current National Institute for Health and Clinical Excellence guidance (neonatal jaundice) is to use IVIG when the serum bilirubin continues to rise at more than 8.5 µmol/l/h in spite of phototherapy, although further research is recommended.
Exchange transfusion in HDN is required for:
- •
severe anaemia: haemoglobin <10 g/dl at birth (with the possible exception of anti-Kell, as mentioned above)
- •
severe or rapidly increasing hyperbilirubinaemia
- •
signs of bilirubin encephalopathy.
Details of the product to use for exchange transfusion are given on p. 782 and are summarised in the current .
‘Late’ anaemia presents at a few weeks of age in some babies with milder haemolytic disease who do not require exchange transfusion and in babies who have had earlier exchange transfusion. The blood film shows evidence of ongoing haemolysis and the anaemia is aggravated by the normal postnatal suppression of erythropoiesis. Such babies may require ‘top-up’ transfusion for symptomatic anaemia; conventional guidelines for neonatal transfusion can be followed (see Table 30.13 ) but irradiated blood must be used for infants previously receiving intrauterine transfusion, to prevent the risk of transfusion-associated graft-versus-host disease (TA-GVHD) ( ). Thus, all babies found to have a strongly positive DAT at birth and all treated by intrauterine transfusion must be followed up to monitor the rate of haemoglobin fall. It is important to note that administration of prophylactic antenatal anti-D to rhesus D-negative mothers quite commonly causes a weakly positive DAT in the baby at birth. Provided there is a clear history of antenatal prophylaxis and the Hb and the blood film are normal, it is not necessary to arrange further investigation and follow-up of such babies. It is worth noting that babies with ABO HDN rarely require ‘top-up’ transfusion unless severe anaemia in the first few days of life (Hb <10 g/dl) has been a feature. Where the haemoglobin is falling more rapidly than normal, particularly in babies with unconjugated hyperbilirubinaemia, monitoring must be continued until the jaundice resolves and the haemoglobin reaches a plateau. This may take 8 weeks and it may be helpful to ask the haematologist to review the blood films each time, to look for evidence of ongoing haemolysis. Folic acid (500 µg/kg/day) should be given to all babies with haemolytic anaemia until 3 months of age; folic acid is not necessary for babies with ABO HDN who are not anaemic. Erythropoietin has been used to prevent the need for ‘top-up’ transfusion for late anaemia; both failures and successes of this approach have been reported and erythropoietin is unlikely to prevent the need for transfusion where ongoing haemolysis is brisk ( ; ).
Neonatal haemolytic anaemia due to red cell membrane disorders
A number of red cell membrane disorders may present in the neonatal period. The three most common types of presentation are:
- 1
unexplained haemolysis with jaundice but usually only moderate anaemia (usually due to hereditary spherocytosis)
- 2
as an incidental finding on a routine blood film in the absence of unusual jaundice or anaemia (usually hereditary elliptocytosis)
- 3
severe, transfusion-dependent haemolytic anaemia with a characteristic very low MCV of 50–60 fl (usually due to hereditary pyropoikilocytosis (HPP)).
The main clues that a neonate has a red cell membrane disorder are a family history, otherwise unexplained haemolysis and an abnormal blood film. Red cell membrane disorders can nearly always be recognised by the characteristic shape of red cells on a blood film. However, the identification of the exact type of membrane abnormality is more complex and requires specialised investigations on both the neonate and the parents and close liaison with a haematologist. The osmotic fragility test has largely been replaced by the dye-binding test which is carried out on a small sample of peripheral blood but may need to be performed in a specialised centre ( ; ). It is important to carry out these definitive diagnostic investigations (red cell membrane studies) on pretransfusion blood samples to minimise diagnostic confusion due to transfused cells and to check the blood count and film of both parents if possible ( ).
A brief summary of the three main clinical disorders presenting in neonates is given below; for more detailed information, the reader is referred to comprehensive reviews ( ; ; ).
Hereditary spherocytosis
This is the commonest red cell membrane defect. It occurs in 1 in 5000 live births to parents of northern European extraction, but is less frequently seen in other ethnic groups ( ). It is autosomal dominant, but around 25% of cases are sporadic due to new mutations. Hereditary spherocytosis is genetically heterogeneous – mutations in spectrin, ankyrin, protein 4.1 and protein 3 have all been reported ( ). The usual presentation of hereditary spherocytosis in the neonate is with unconjugated hyperbilirubinaemia. Most affected neonates are not anaemic, but a small proportion have anaemia severe enough to require transfusion. The blood film in hereditary spherocytosis shows moderate numbers of spherocytes; the appearance is identical to that of ABO haemolytic disease, but the two disorders are distinguishable by the negative DAT in hereditary spherocytosis. While some babies will require one or two transfusions during the first 1–2 months of life, very few remain transfusion-dependent after this time and it is important to stop transfusions to evaluate the nadir haemoglobin reached. All children with hereditary spherocytosis should also receive folic acid supplementation (from 500 µg/kg/day in the first 6 months of life and a total daily dose of 2.5 mg from 6 months until 10 years of life, increasing to 5 mg daily thereafter). Splenectomy almost invariably induces complete remission of haemolytic anaemia (and therefore the need for folic acid prophylaxis) but is only indicated for more severely affected children and virtually always deferred until after the age of 6 years.
Hereditary elliptocytosis
This is a more complex disorder. It is caused by different mutations in the genes for spectrin, ankyrin or protein 4.1 ( ; ). In the common, autosomal dominant form of hereditary elliptocytosis, the heterozygotes have no clinical manifestations (i.e. no anaemia and no jaundice) apart from elliptocytes on the blood film. No treatment is required and folic acid prophylaxis is unnecessary, as folate deficiency is not a feature of this condition. Neonates who are homozygous or compound heterozygotes for hereditary elliptocytosis mutations have severe haemolytic anaemia; the most common form is HPP.
Hereditary pyropoikilocytosis
Neonates with HPP have more than one mutation in a red cell membrane protein (they may be homozygous or compound heterozygotes) ( ; ). HPP is uncommon but is important because it causes severe, transfusion-dependent haemolytic anaemia which does not improve with age. The diagnosis of HPP should easily be made by examining blood films from the baby (which shows lots of bizarre fragmented red cells and microspherocytes) and both parents (one or both often have red cell elliptocytosis); a useful diagnostic clue is the low MCV at birth (<60 fl). Red cell transfusion is usually necessary until the child is old enough to undergo splenectomy, to which there is an excellent response.
Neonatal haemolysis due to red cell enzymopathies
The principal red cell enzymopathies which present in the neonatal period are glucose-6-phosphate dehydrogenase (G6PD) deficiency and pyruvate kinase (PK) deficiency. They usually present with unconjugated hyperbilirubinaemia; clinically, they are indistinguishable from red cell membrane disorders, although anaemia is uncommon in neonates with G6PD deficiency. Unlike with the membrane disorders, there are often no diagnostic changes on the blood film.
Glucose-6-phosphate dehydrogenase deficiency
G6PD deficiency is seen in all ethnic groups but has a high prevalence in individuals from central Africa (20%) and the Mediterranean (10%). The G6PD gene is on the X chromosome and therefore most affected neonates are boys, although female carriers may sometimes be identified as a result of neonatal jaundice screens. In neonatal G6PD deficiency, jaundice usually presents within the first few days of life and is often severe; anaemia is extremely rare and the blood film is completely normal, thus the diagnosis must be made by assaying G6PD on a peripheral blood sample ( ). It is not clear why some, but not all, G6PD-deficient neonates develop neonatal jaundice. In addition, the pathogenesis of the jaundice is also unclear, since most babies with G6PD deficiency have no evidence of haemolysis. The most important management issues in neonatal G6PD deficiency are close monitoring of the bilirubin, particularly where interactions with other risk factors for neonatal hyperbilirubinaemia are present, such as Gilbert syndrome or hereditary spherocytosis, since kernicterus has been reported in this setting ( ; ), and counselling parents of affected babies about which medicines, chemicals and foods may precipitate haemolysis ( Table 30.6 ). If exchange transfusion is required for severe hyperbilirubinaemia, conventional guidelines for exchange transfusion can be followed (see Chs29.2 , 44 ). Certain uncommon variants of G6PD deficiency are associated with chronic haemolysis, and for these children folic acid supplements should be given ( ). However, for the vast majority of patients there is no chronic haemolysis and no anaemia and therefore folic acid supplements are not indicated.
Antimalarials |
Antibiotics |
Analgesics |
|
Chemicals |
|
* Acceptable in acute malaria.
† Some sulphonamides do not cause haemolysis in most G6PD-deficient patients, e.g. sulfadiazine.
‡ To be avoided in some types of G6PD deficiency (can be taken by patients with the common, African A-form of G6PD deficiency).
Pyruvate kinase deficiency
PK deficiency is the second most common red cell enzymopathy in neonates. It is autosomal recessive and clinically heterogeneous, varying from anaemia severe enough to cause hydrops fetalis to a mild unconjugated hyperbilirubinaemia ( ; ). In severe cases. the jaundice has a rapid onset within 24 hours of birth and exchange transfusion may be required ( ). The diagnosis is made by measuring pretransfusion red cell PK activity; in mild cases, the PK activity may be relatively modestly reduced, making the diagnosis difficult, and it is often useful to assay levels in the parents for confirmation. The blood film is sometimes distinctive but more often shows non-specific changes of non-spherocytic haemolysis and therefore it is good practice to assay PK in all babies with unexplained haemolysis after the common causes have been excluded. Management in the neonatal period depends on the severity of the jaundice and anaemia; some, but not all, children are transfusion-dependent and folic acid supplements should be given to prevent deficiency due to chronic haemolysis.
Other red cell enzymopathies presenting in neonates
The other red cell enzymopathies are rare. The most important to be aware of in the neonatal period is triosephosphate isomerase deficiency, which is autosomal recessive ( ). One-third of cases present with neonatal haemolytic anaemia and this may be the only presenting feature at this age; the devastating neurological features of this disorder only become apparent 6–12 months later ( ). Persistent haemolysis should therefore always be investigated.
Neonatal haemolysis due to haemoglobinopathies
The haemoglobinopathies, with the exception of α-thalassaemia major, do not usually present in the neonatal period. Occasional non-thalassaemic, structural α-globin and γ-globin gene mutations, which are clinically completely silent in adults and children, cause transient haemolytic anaemia (and diagnostic confusion!) in the neonate (see below). Symptoms and signs of the major β-globin haemoglobinopathies (sickle-cell disease and β-thalassaemia major) are rare in neonates, although modern techniques (e.g. high-performance liquid chromatography (HPLC), isoelectric focusing) allow the diagnosis to be made on neonatal blood samples where family studies indicate that both parents are carriers ( ). Many countries and regions with a high prevalence of haemoglobinopathies, including the UK, have neonatal screening programmes to facilitate early diagnosis, which is particularly important in sickle-cell disease in order to start penicillin prophylaxis as soon as possible ( ; ; ).
Alpha-thalassaemia major
Alpha-thalassaemia major occurs when all four α-globin genes on chromosome 16 are deleted ( ). It predominantly affects families of south-east Asian origin and presents with mid-trimester fetal anaemia or hydrops fetalis which is fatal within hours of delivery (occasional babies have lived a few days). The only long-term survivors of α-thalassaemia major are those who received intrauterine transfusions ( ; ; ). In recent years there have been several reports of normal growth and development where intrauterine transfusion is commenced during the second trimester ( ; ); however, there is a high incidence of hypospadias in boys and other survivors have limb defects and/or severe neurological problems ( ; ). If intrauterine transfusions are delayed until the anaemia is severe, neonatal pulmonary hypoplasia is a cause of early mortality. The diagnosis of α-thalassaemia major should be suspected in any case of severe fetal anaemia presenting in the second trimester and any case of hydrops fetalis with severe anaemia in which the parents come from south-east Asia (it is also seen occasionally in families who originate from India, the Middle East or the Mediterranean). Checking the blood counts of the parents will immediately identify whether they are at risk of having a child with α-thalassaemia major – both parents will be carriers of a chromosome 16 in which both of the two α-globin genes are deleted and so they will have hypochromic, microcytic red cell indices (MCV usually <74 fl and MCH usually <24 pg). The diagnosis of α-thalassaemia major is confirmed by haemoglobin electrophoresis or HPLC (which shows only Hb Barts and Hb Portland; HbF and HbA are absent); the blood film shows hypochromic, microcytic red cells with vast numbers of circulating nucleated red cells. Neonatal management of α-thalassaemia major has no impact on survival unless the baby has received intrauterine transfusions; for these transfused neonates, management is the same as for β-thalassaemia, i.e. lifelong red cell transfusions or bone marrow transplantation after the age of 2 years ( ; ).
Alpha- and gamma-globin chain structural abnormalities
Most α- and γ-globin gene variants are clinically silent. Occasional α-globin gene variants may cause haemolytic anaemia in the newborn because when the abnormal α-globin associates with γ-globin the resultant haemoglobin is unstable whereas when the variant α-globin associates with β-globin the resultant haemoglobin is stable. An example of this is Hb Hasharon: α 214Asp→His -γ 2 is unstable; but as γ-globin chain production is physiologically switched off and β-globin chain production predominates, the α 214Asp→His -β 2 produced is stable and the haemolytic anaemia completely resolves ( ). A similar principle occurs in the γ-globin variant HbF-Poole, which causes neonatal haemolytic anaemia that resolves as the switch from γ- to β-globin occurs ( ). These variants can be identified by haemoglobin HPLC and are worth considering when commoner causes of haemolysis have been excluded.
Beta-thalassaemias and sickle-cell disease
Although these disorders are asymptomatic in neonates, if they are identified as a result of neonatal screening programmes, specialist advice should be sought as soon as possible. Babies with sickle-cell disease (homozygous sickle-cell disease, SC disease or S-β-thalassaemia) should be started on prophylactic penicillin V (62.5 mg twice daily) and folic acid (500 µg/kg/day) ( ). Babies with β-thalassaemia major usually start to require transfusion around the age of 6 months but benefit from folic acid supplementation until regular transfusions begin ( ).
Anaemia due to blood loss
Blood loss causing neonatal anaemia may be very obvious, e.g. a large subgaleal haematoma or rupture of the cord, or be concealed and easy to miss unless specifically sought (e.g. fetomaternal bleeds). Conventionally, the causes of anaemia due to blood loss are classified according to the timing of the blood loss – during fetal life, at the time of delivery or postnatally (see Table 30.4 ). In neonates admitted to hospital the most common cause of anaemia is blood loss secondary to iatrogenic blood letting.
Blood loss prior to birth
Twin-to-twin transfusion
Twin-to-twin transfusion occurs in monochorionic twins with monochorial placentas ( Ch. 23 ) ( ). Bleeding may be acute, particularly during the second stage of labour, or chronic ( ). Chronic twin-to-twin transfusion can cause a marked difference in birthweight between twins, although recent studies show that the majority of twin pairs have a discordance in haemoglobin of <5 g/dl ( ). The diagnosis is now usually made in fetal life and antenatal treatment may be required ( Chs9 , 23 ). The donor twin is smaller and may be pale and lethargic or have overt cardiac failure; the recipient twin may be plethoric, with hyperviscosity and hyperbilirubinaemia and may rarely have a haemoglobin as high as 30 g/dl. Where the haemoglobin/haematocrit are very high, disseminated intravascular coagulation (DIC) can occur. Management of DIC is described on page 776 , and that of polycythaemia on page 767 .
Fetomaternal haemorrhage
This may occur spontaneously or secondary to trauma, such as road traffic accidents or falls. Most spontaneous fetomaternal bleeds occur in the third trimester or during labour. Fetomaternal bleeds may also be increased by invasive procedures such as fetal blood sampling and caesarean section. The degree of anaemia is variable and the clinical presentation depends on the amount and rate of blood loss. Most episodes involve very small quantities of blood (0.5 ml or less) but acute loss of >20% of the blood volume may cause intrauterine death, circulatory shock or hydrops ( ). Diagnostic clues are anaemia at birth in an otherwise well term baby with no or minimal jaundice. The most useful diagnostic tests are a DAT to exclude immune haemolysis, a reticulocyte count to exclude red cell aplasia, a Kleihauer test on maternal blood to quantify the number of HbF-containing fetal RBCs in the maternal circulation and a blood film ( ). Where the baby has bled acutely just prior to delivery, the blood film is normochromic/normocytic with large numbers of nucleated red cells. In this situation, the haemoglobin may be normal at delivery but fall rapidly as haemodilution occurs. Where there is chronic blood loss, the baby is often well but may present with cardiac failure; the blood film in this situation is hypochromic/microcytic, the nucleated red cells are less prominent and the Kleihauer result may be difficult to interpret. Another point of note is that, where there is ABO incompatibility between the mother and baby, the fetal cells may be rapidly destroyed within the maternal circulation – a high index of suspicion of fetomaternal haemorrhage as a cause of neonatal anaemia is needed because it is important to perform the Kleihauer test as soon as possible, to increase the chance of detection of fetal cells. In many cases, an acute fetomaternal bleed supervenes upon chronic fetomaternal blood loss. In this situation, severe anaemia (Hb <5 g/dl) has been shown to confer a poor prognosis, as most survivors have evidence of brain injury, although there are notable exceptions ( ).
Blood loss at or after delivery
Blood loss around the time of delivery is usually due to obstetric complications, including placenta praevia, placental abruption or incision of the placenta during caesarean section. Blood loss in this situation is from the maternal circulation, although the fetus can be subject to hypoxia as a result. Rarely, a vasa praevia is present, and is inevitably torn when the membranes rupture, leading to severe fetal blood loss. This can easily be missed because the volume of blood is relatively small in obstetric terms and it is not possible to distinguish fetal from maternal blood by eye. The incidence of vasa praevia is estimated to be around 1 : 2500 and the condition carries a high fetal mortality. The presence of a vasa praevia can be detected antenatally with a colour Doppler vaginal ultrasound but this is not routine practice; if a vasa praevia is found then the baby should be delivered by caesarean section before membrane rupture.
Haematological changes occur after massive internal bleeding in the baby, e.g. subaponeurotic or retroperitoneal bleeding, and may be particularly severe where there is damage to the liver. While most cases of internal bleeding are associated with traumatic delivery, it is important to search for any underlying bleeding diathesis in such babies, particularly haemophilia A or B and vitamin K deficiency. Inherited and acquired coagulation disorders that may present with bleeding at birth or during the neonatal period are discussed on pages 775–776 .
Anaemia of prematurity
Pathogenesis
The haemoglobin falls after birth in all newborns, regardless of gestational age. This normal physiological fall in haemoglobin is greater in preterm than in term neonates and has been termed ‘physiological anaemia of prematurity’ since it does not appear to be associated with any abnormalities in the baby. The pathogenesis is not fully elucidated but contributory factors include the reduced red cell lifespan of fetal erythrocytes, the relatively low erythropoietin concentration and the rapid growth rate ( ). In practice, perhaps because of routine supplementation of preterm neonates with folic acid and iron, nutritional deficiency rarely plays a role ( ; ; ). On the other hand, for the majority of preterm infants in hospital, iatrogenic blood letting for diagnostic tests contributes to this physiological process. The clinical significance of anaemia of prematurity is mainly that the need for ‘top-up’ transfusion in preterm infants can be reduced if clinicians are aware of the normal nadir in erythropoiesis in neonates and the available measures to prevent the anaemia becoming severe.
Diagnosis
In a well term infant, the nadir in haemoglobin is as low as 9.4–11 g/dl and occurs at 8–12 weeks of age (see Table 30.2 ); for a preterm infant, the nadir in haemoglobin occurs earlier (4–8 weeks of age) and is lower (6.5–9 g/dl). The diagnosis is usually straightforward – a well preterm baby has a slowly falling haemoglobin with a completely unremarkable blood film showing normochromic/normocytic red cells, slightly low reticulocytes (20 × 10 9 /l) and no nucleated red cells.
Management
There are three facets to the management of anaemia of prematurity. The first is to exclude other causes of anaemia using clinical features and a diagnostic algorithm (see p. 762 , Fig. 30.1 ). Secondly, a decision whether or not to transfuse should be made (the indications and principles of neonatal transfusion are discussed in detail on pp. 782–785 ). Thirdly, with increasing recognition of potential transfusion hazards, amelioration of neonatal anaemia to reduce the need for transfusion has become extremely important.
The role of erythropoietin and haematinics
The severity of anaemia of prematurity and thereby the need for red cell transfusion can be reduced by a combination of the following approaches:
- •
limiting iatrogenic blood loss by appropriate use of blood tests ( )
- •
iron and folate supplementation for all preterm infants:
iron 3 mg/kg/day from 4–6 weeks of age (a pragmatic approach is to give 1 ml of sodium ironedetate (Sytron) once daily) or iron-fortified formula with 0.5–0.9 mg/dl iron ( ; ; )
folic acid 50 µg daily or 500 µg once weekly ( )
- •
judicious use of erythropoietin ( ; ).
The many controlled trials of erythropoietin for prevention of neonatal anaemia have been extensively reviewed ( ), including two meta-analyses ( ; ), and are only briefly summarised here. Recombinant erythropoietin is biologically effective in that it stimulates erythropoiesis in all preterm infants and there is no evidence of erythropoietin insensitivity. Erythropoietin is also able to reduce red cell transfusion requirements in preterm infants ( ; ; ; ; ). However, in most studies there is no evidence to support the clinical effectiveness of erythropoietin, i.e. that it reduces the number of transfusions to an extent which demonstrably reduces the hazards of transfusion. At best, the studies show that erythropoietin reduces the number of transfusions in relatively well infants with low transfusion requirements ( ; ; ; ). One study has shown that the use of erythropoietin together with careful nutritional supplementaion and judicious use of laboratory tests to reduce iatrogenic blood loss can halve the transfusion requirements in a cohort of sick extremely-low-birthweight neonates ( ). However, most studies have shown that, even in high doses, the erythropoietin-mediated increase in red cell production is unable to increase sufficiently to cope with the need for frequent phlebotomy and multiple transfusions in sick preterm infants ( ; ). Despite its marginal role in reducing transfusion requirements, erythropoietin may become more important again if worries over the safety of blood transfusion lead to reduced availability and parental acceptance of red cell transfusion. At present, the main therapeutic roles for erythropoietin in neonates are:
- •
in preventing anaemia in infants who have received intrauterine transfusions for alloantibody-mediated anaemia ( )
- •
in a non-emergency situation where red cell transfusions are against the parents’ wishes and are not felt to be absolutely essential to save the life of the baby (e.g. preterm babies of Jehovah’s witnesses) ( ).
An effective dose of recombinant erythropoietin in this setting is 300 µg/kg as a single subcutaneous injection three times per week starting in the first week of life. Epoetin-β should be used in view of the potential risk of red cell aplasia described (in adults) with epoetin-α, the alternative form of recombinant erythropoietin ( ). This is because the haemoglobin does not start to rise until about 10–14 days after erythropoietin has been commenced. In addition, iron supplements (Sytron, as above) should be started as soon as possible, to prevent the rapid development of iron deficiency in erythropoietin-treated infants (the dose may need to be increased up to a maximum of 9 mg/kg if iron deficiency develops on the standard dose of Sytron) ( ).
A simple diagnostic approach to neonatal anaemia
Red cell disorders associated with neonatal or fetal anaemia present in three main ways: with a low haemoglobin (anaemia), with jaundice due to haemolysis or with hydrops. Table 30.4 lists the most common causes of anaemia, and the most common haematological causes of jaundice and hydrops are shown in Table 30.7 and Table 30.5 , respectively. A diagnostic algorithm to help identify the most likely cause of neonatal hyperbilirubinaemia and the investigations which are most useful is shown in Figure 30.2 . This is based on simple observations and simple tests available in almost all haematology laboratories. When in doubt about the best investigations to use and the interpretation of the results, discussion with a haematologist at an early stage should be helpful.
Immune |
|
Red cell membrane disorders |
|
Red cell enzymopathies |
|
Haemoglobinopathies |
|
Infection |
|
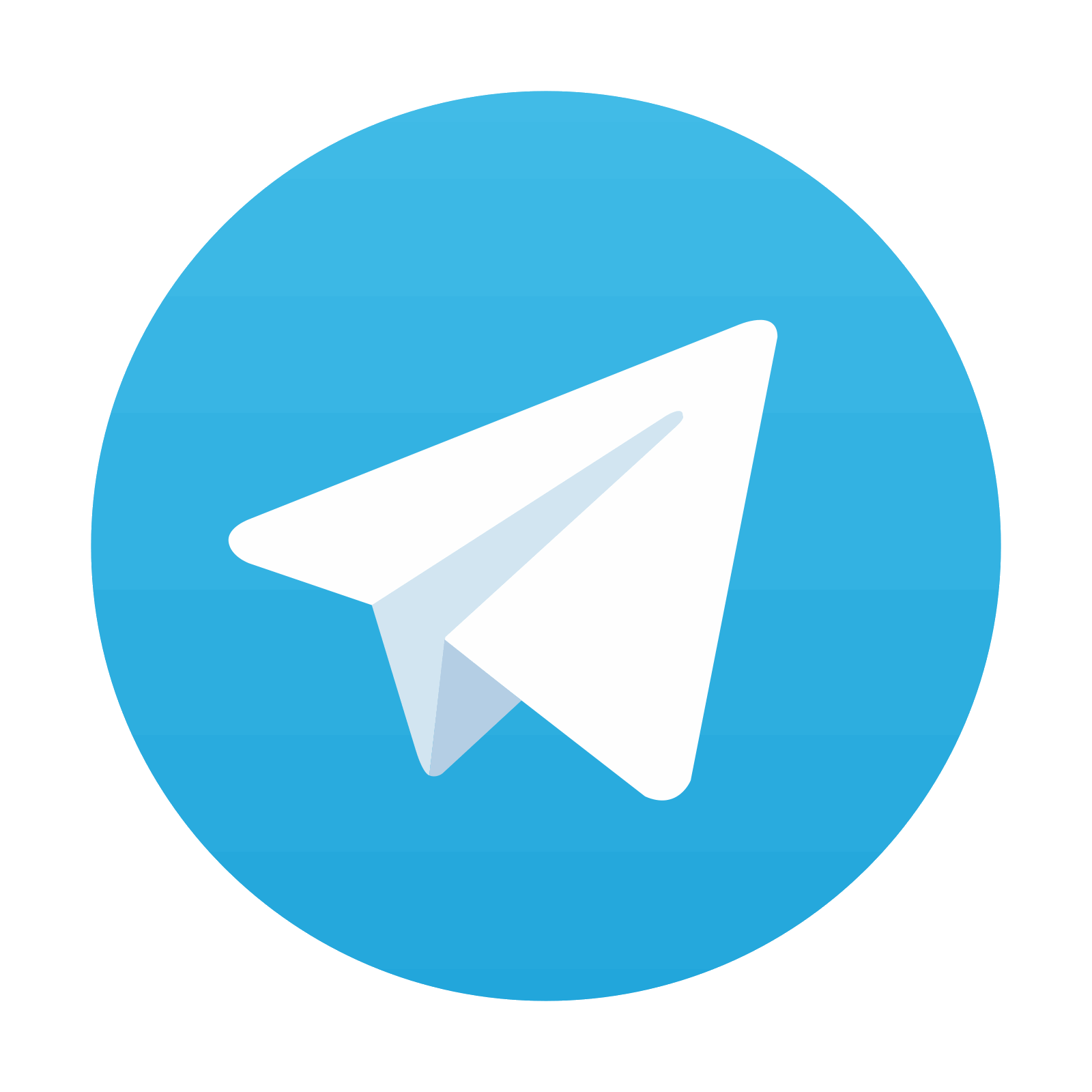
Stay updated, free articles. Join our Telegram channel
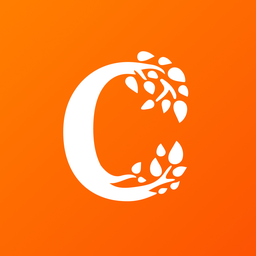
Full access? Get Clinical Tree
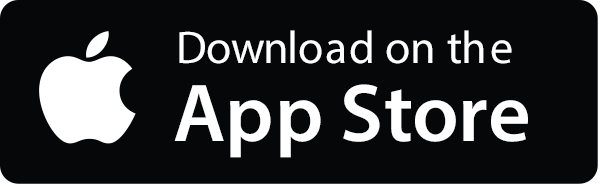
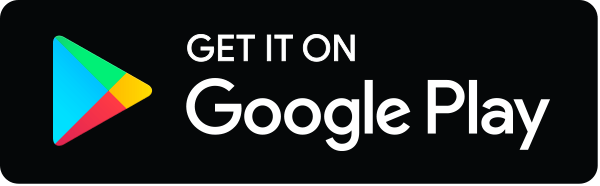