Objective
Synthetic glucocorticoid administration to women threatening preterm delivery increases neonatal survival. However, mounting evidence shows that fetal exposure to glucocorticoid levels higher than appropriate for current maturation adversely programs offspring development. We examined fetal synthetic glucocorticoid multigenerational metabolic effects on F1 and F2 female offspring.
Study Design
At 0.7 gestation, pregnant F0 ewes received 4 injections of dexamethasone (2 mg, approximately 60 ug.kg −1 day −1 12 hours apart) or saline (control). F1 female offspring were bred to produce F2 female offspring. Postpubertal pancreatic β-cell function was tested in F1 and F2 by intravenous glucose tolerance test.
Results
F1 and F2 ewe lambs showed reduced birthweight and morphometrics, and similar increased fasting glucose and decreased intravenous glucose tolerance test β-cell response.
Conclusion
This is the first demonstration of multigenerational programming of later life β-cell response by clinically relevant doses of synthetic glucocorticoid indicating the need for study of long-term effects of fetal exposure to synthetic glucocorticoid.
The ground-breaking demonstration by Liggins of glucocorticoid (GC) induced acceleration of fetal lung development in sheep led to a clinical trial showing decreased morbidity and mortality in premature infants whose mothers received synthetic GC. It is now routine practice to treat women threatening preterm delivery before 34 weeks’ gestation with GC. Although treatment with synthetic GC confers great benefit by lowering neonatal mortality and morbidity, evidence is mounting that in addition to the major benefits of accelerated lung maturation, fetal exposure to GC levels higher than those appropriate for the current stage of fetal maturation produces intrauterine growth restriction (IUGR) in several species, sheep, nonhuman primates, and humans altering the developmental trajectory of many fetal organ systems with potential for adverse developmental programming effects in later life. These similarities across species suggest common underlying mechanisms producing adverse outcomes. Animal studies demonstrate that delayed offspring endocrine, renal, and metabolic effects emerge later in life with the potential to predispose to chronic disease. The nature and extent of these long-term effects needs to be understood.
Fetal programming can be defined as— a response to a specific challenge during a critical fetal time window that alters development with persistent effects on offspring phenotype . Increases in fetal and maternal GC have been demonstrated to occur in response to many challenges that result in fetal programming. In addition, offspring outcomes resulting from challenges such as poor maternal nutrition can be prevented by inhibiting GC changes occurring in response to the challenge. As a result, many investigators consider exposure to excessive endogenous GC levels at critical fetal developmental periods to be a common causative factor of many, though not necessarily all, fetal programming responses to challenges during development, eg, maternal stress and poor nutrition. One leader in the field writes, “similarities in phenotype associated with dietary and glucocorticoid exposures have led to suggestions that they may act through a shared mechanism …”
The only multigenerational study on effects of fetal exposure to GC was conducted in rodents—an altricial species with a very different profile of fetal development to precocial species such as humans and sheep. The paradigm was very different from the human clinical treatment regimen and involved exposure continuously for 6 days—approximating 30% of the whole of gestation. In addition, that study involved male offpring. To enable translation of any fetal programming effects of synthetic GC on insulin and glucose metabolism after human fetal exposure, it is necessary to conduct studies in precocial species with dosing protocols that produce exposures that equal the duration, dose, and critical developmental window of exposure experienced during human synthetic GC therapy in preterm labor.
We hypothesized that exposure of female fetal lambs (F1 generation) to synthetic GC administered to their mothers (F0 generation) at 27 weeks’ human gestation equivalent would have effects in F1 and F2 female offspring on metabolic phenotype as reflected by birthweight, morphometric measurements, postnatal growth patterns, and glucose and insulin dynamics. To test this hypothesis, we administered dexamethasone (DEX) to pregnant ewes at 103 and 104 days’ gestation (term 150 days) and determined F1 and F2 female offspring outcomes.
Materials and Methods
Care and use of animals
All procedures were approved by the University of Wyoming Animal Care and Use Committee. Twenty-two F0 nulliparous Rambouillet X Columbia crossbred ewes (approximately 16 months of age) were used to produce the F1 and F2 ewe lambs studied. F0 ewes were bred to a single ram in 1 of 2 consecutive years. After natural mating, ewes were fed in accordance with National Research Council (NRC) maintenance requirements. On days 103 and 104 of gestation, ewes averaged 73.6 ± 4.0 kg (mean ± SEM) body weight and were randomly assigned to 1 of 2 treatment groups: DEX ewes (n = 10) received 4 injections of 2 mg of DEX (intramuscular [im]; Vedco, St. Joseph, MO) 12 hours apart, a dose equal to approximately 60 μg.kg −1 : day −1 . Control ewes (n = 12) received equivalent volumes of saline im. Ewes were allowed to lamb naturally. At lambing, F1 newborn body weight and morphometrics were recorded on all female lambs. There were 8 control twins (2 sets were both females) and 4 control singles. In the DEX lambs, there were 7 twins (3 female twin sets) and 3 singles. After lambing, all F0 ewes were given free choice access to alfalfa hay. Before 2 weeks of age, F1 female lambs were tail-docked as per Federation of Animal Science Societies’ recommendations. Two control and 3 DEX female F1 lambs all from above mentioned twin births died of natural causes before 3 months of age and their data was excluded from this study. The study was conducted on n = 10 DEX and 12 control F1 offspring. F1 ewe lambs were given free access to a standard commercially available creep feed (Lamb Creep B30 w/Bovatec; Ranch-way Feeds, Ft. Collins, CO) from birth to weaning at 4 months of age and placed in an outdoor housing facility with shelter and ad libitum water. These F1 ewe lambs after weaning were maintained in accordance with NRC requirements for replacement ewes. Diet consisted of alfalfa and corn to meet NRC requirements with ad libitum access to a trace mineral salt block. Diets were adjusted up for weight gain every month. Lamb body weights and ponderal index (lamb body weight, kg/[lamb height/m 3 ]) were recorded at postnatal day 7, 14, 28, and then monthly until 12 months of age.
F1 ewe lambs received maintenance requirements until either 16 or 28 months of age depending on the year in which they were born. F1 ewes weighed 65.1 ± 3.5 (n = 12) and 64.7 ± 3.7 kg (n = 10) control and DEX, respectively, when naturally mated to a single ram with mating date noted using a marking ram harness. Numbers of lambs born per F1 ewe were recorded as well as their birthweight and morphometrics. All F2 ewe lambs (n = 9, DEX and 8, control) were fed in a similar fashion as their F1 mothers and postnatal body weights were recorded at the same times. There were 3 control F2 twin sets (all male-female sets) and 5 control F2 singles. In the DEX F2 group, there were 4 twin sets (1 female twin set and 3 male-female twin sets) and 4 singles.
Investigators were blinded to treatment groups. For the study of F1 females, a random subsample (chosen by random number draw) of 6 per treatment group of female F1 offspring at 2.5 or 3.5 year of age (3 per age and birth type [twin or single] in each treatment group). For the F2 study, 6 females whose grandmothers received DEX and 6 whose grandmothers received saline randomly selected at 6 months of age (3 per birth type in each treatment group) were chosen for study. A catheter (Abbocath, 16 ga; Abbott Laboratories, North Chicago, IL) was placed aseptically into the jugular vein 24 hours before the start of the intravenous glucose tolerance test (IVGTT) blood sampling. Catheters were sutured to the skin to secure them and an extension set (Seneca Medical, Tiffin, OH) attached for undisturbed infusion and sampling. The neck and shoulder area were covered with netting (Surgilast Tubular elastic dressing retainer, Derma Science Inc, Princeton, NJ) to prevent catheter damage. F1 ewes and F2 lambs were maintained in neighboring individual pens with free access to water. No feed was provided for ∼18 hours before and during the IVGTT, which has been described in detail. Jugular blood samples (∼6 mL) were obtained into chilled tubes (heparin plus NaF; 2.5 mg/mL; Sigma-Aldrich, St. Louis, MO) at −15, and 0 minutes relative to a 0.25 g/kg intravenous bolus infusion of 50% dextrose solution (Vedco, St, Joseph, MO) over 5 seconds. Blood samples were collected at 2, 5, 10, 15, 20, 30, 45, 60, 90, and 120 minutes after dextrose infusion. All blood samples were immediately placed on ice, centrifuged at 2500 × g and plasma was collected and stored at −80°C. F1 and F2 offspring were given free access to pelleted alfalfa after the IVGTT and throughout the rest of the challenges.
Biochemical and hormone assays
Glucose was measured colorimetrically in triplicate (Liquid Glucose Hexokinase Reagent; Pointe Scientific, Inc, Canton, MI) as previously described. Mean intraassay coefficient of variation (CV) was 1.2% and interassay CV was 2.8%. Insulin was measured in duplicate by commercial radioimmunoassay (RIA) (Coat-A-Count Insulin RIA; Siemens Medical Solutions Diagnostics, Los Angeles, CA) with intra- and interassay CV of 8.9% and 13.2%, respectively, and a sensitivity of 2.6 μIU/mL.
Statistical analysis
All data were normally distributed and were not transformed. Data are presented as least square means ± SEM, and differences considered significant at P ≤ .05, with a tendency set at P ≤ .10. Graphpad Prism (GraphPad Software Inc, La Jolla, CA) was used to calculate the area under the curve (AUC) for plasma glucose and insulin response curves during the IVGTT. Baseline concentrations of glucose and insulin in all samples before infusion were averaged to give baseline concentrations. An insulin to glucose ratio was calculated from the baseline values and using the AUC from the IVGTT. Plasma glucose and insulin during the IVGTT were analyzed as repeated measures using MIXED procedure of SAS (SAS Institute Inc, Cary, NC) with treatment and time, and their interaction in the model. AUC and fasting concentrations of glucose and insulin, birthweights and morphometrics, gestation lengths, number of lambs per ewe, and the baseline and AUC insulin to glucose ratios were analyzed using the GLM procedure of SAS with treatment in the model. Body weights of both F1 and F2 offspring both before 2 months of age and from 3 to 12 months of age were analyzed as repeated measures using MIXED procedure of SAS with treatment, date, and their interaction in the model. F1 ewe age (1 or 2 years) was initially included in all F1 ewe models but was found to be nonsignificant ( P < .46) and was therefore removed from the models. Birth type (twin vs single) was initially included in all models but was found to be nonsignificant ( P < .16) and was therefore removed. Data are provided as mean ± SEM throughout.
Results
Pregnancy and growth
Gestation length was 3 days shorter in the DEX F0 ewes compared with the control F0 ewes ( P < .05; 152.3 ± 0.5 vs 155.4 ± 0.5, respectively). The duration of pregnancy in DEX F1 offspring tended to be shorter than their corresponding controls by almost 2 days, though this difference did not quite reach significance (147.6 ± 0.7 vs 149.3 ± 0.06, respectively, [ P < .06]). There was no difference in the number of lambs delivered by each F0 ewe (1.67 ± 0.14 vs 1.70 ± 0.15) or F1 ewe (1.16 ± 0.13 vs 1.40 ± 0.14, control and DEX, respectively). Birthweights and morphometrics for F1 and F2 offspring are shown in the Table . Female F1 offspring of DEX treated ewes had reduced birthweights, crown rump lengths, and thoracic circumference compared with control F1 female newborn lambs. Interestingly, DEX F2 newborn lambs also had reduced birthweight, crown rump length, thoracic, and, additionally, abdominal circumference was reduced compared with F2 control newborn lambs ( Table ). Ponderal index in both DEX F1 and F2 offspring compared with their corresponding control offspring ( Table ). From day 7 to 56 of age ( Figure 1 , A) there was a treatment * day interaction, with body weight being less in F1 DEX than F1 control lambs at days 21 to 56. From 3 to 12 months of age ( Figure 1 , B) DEX F1 lambs weighed less than control F1 lambs. Growth patterns of the F2 female lambs are shown in Figure 2 . From days 7 to 56 of age ( Figure 2 , A), DEX F2 lambs weighed less than control F2 lambs. There was a treatment * month interaction with DEX F2 female lambs having reduced body weights from 3 to 5 months of age ( Figure 2 , B), then returning to a similar body weight from 6 to 8 months of age. Body weights of DEX F1 ewes at catheterization for the IVGTT were less ( P < .05) than control F1 ewes (F1 65.1 ± 2.5 vs 72.2 ± 2.5 kg, respectively). Body weights of DEX F2 lambs at catheterization were less ( P < .05) than control F2 lambs (44.8 ± 2.4 vs 51.2 ± 2.4 kg, respectively).
Variable V F1 offspring | Control | DEX | P value |
---|---|---|---|
n | 12 | 10 | |
Birthweight, kg | 5.59 ± 0.17 | 5.03 ± 0.18 | .04 |
Crown rump length, cm | 57.6 ± 1.0 | 53.9 ± 1.0 | .02 |
Ponderal index, kg/m 3 | 29.25 ± 1.00 | 32.12 ± 1.06 | .04 |
Left humerus length, cm | 15.1 ± 0.4 | 14.0 ± 0.5 | .09 |
Right humerus length, cm | 15.0 ± 0.5 | 13.7 ± 0.5 | .06 |
Biparietal distance, cm | 18.8 ± 0.3 | 18.1 ± 0.3 | .09 |
Thoracic circumference, cm | 42.3 ± 0.4 | 40.7 ± 0.4 | .02 |
Abdominal circumference, cm | 40.1 ± 0.7 | 39.9 ± 0.7 | .80 |
F2 offspring | Saline | DEX | P value |
---|---|---|---|
n | 8 | 9 | |
Birthweight, kg | 6.62 ± 0.29 | 5.72 ± 0.26 | .03 |
Crown rump length, cm | 56.0 ± 1.3 | 50.2 ± 1.2 | .01 |
Ponderal index, kg/m 3 | 37.70 ± 1.16 | 45.22 ± 1.33 | .03 |
Left humerus length, cm | 14.2 ± 0.5 | 14.0 ± 0.4 | .68 |
Right humerus length, cm | 14.4 ± 0.5 | 14.2 ± 0.4 | .69 |
Biparietal distance, cm | 15.4 ± 0.5 | 15.0 ± 0.5 | .52 |
Thoracic circumference, cm | 44.3 ± 0.8 | 41.6 ± 0.7 | .02 |
Abdominal circumference, cm | 43.9 ± 0.8 | 40.1 ± 0.8 | .01 |


IVGTT
The plasma glucose and insulin concentrations before and during the IVGTT for F1 female offspring at 2.5 to 3.5 years of age are shown in Figure 3 . Plasma glucose was elevated in DEX F1 offspring at baseline compared with control F1 offspring (62.0 ± 2.5 vs 54.0 ± 2.5 mg/dL, respectively; P = .03). However, there was no difference in fasting baseline plasma insulin between DEX and control F1 offspring (6.59 ± 1.16 vs 7.66 ± 1.16 μIU/mL, respectively). There was a tendency for the fasting baseline insulin to glucose ratio to be reduced in DEX F1 offspring compared with control F1 offspring (0.10 ± 0.02 vs 0.14 ± 0.02, respectively; P = .07). Plasma glucose concentrations were higher ( Figure 3 , A) and AUC for glucose higher in DEX F1 offspring compared with control F1 offspring during the IVGTT. There was also a treatment ⁎ time interaction ( P < .01, Figure 3 , B) for plasma insulin concentration during the IVGTT, with DEX F1 female offspring having reduced plasma insulin concentration at 10 to 45 minutes postglucose administration. As a result, DEX F1 offspring showed a reduced AUC for plasma insulin compared with control F1 offspring. The insulin to glucose ratio for the AUC during the IVGTT were reduced ( P < .01) in DEX F1 offspring compared with control F1 offspring (0.48 ± 0.12 vs 1.07 ± 0.12, respectively).
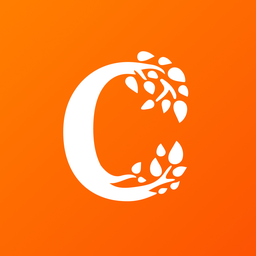
Full access? Get Clinical Tree
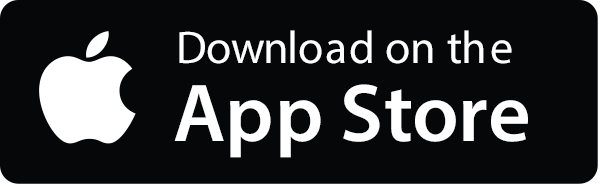
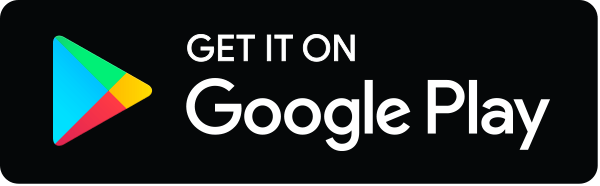