All major external and internal structures are established during the fourth to eighth weeks. By the end of this embryonic period, the main organ systems have started to develop. As the tissues and organs form, the shape of the embryo changes, and by the end of this period, the embryo has a distinctly human appearance. Because the tissues and organs are differentiating rapidly, exposure of embryos to teratogens during this period may cause major birth defects. Teratogens are agents (such as some drugs and viruses) that produce or increase the incidence of major birth defects (see Chapter 20 ).
Phases of Embryonic Development
Human development is divided into three phases, which to some extent are interrelated:
- •
The first phase is growth , which involves cell division and elaboration of cell products.
- •
The second phase is morphogenesis , the development of shape, size, and other features of a particular organ or part or the whole body. Morphogenesis is a complex molecular process controlled by the expression and regulation of specific genes in an orderly sequence. Changes in cell fate, cell shape, and cell movement allow the cells to interact with each other during the formation of tissues and organs.
- •
The third phase is differentiation , during which cells are organized in a precise pattern of tissues and organs that are capable of performing specialized functions.
Folding of Embryo
5
A significant event in the establishment of body form is folding of the flat trilaminar embryonic disc into a somewhat cylindric embryo ( Fig. 5.1 ). Folding occurs in the median and horizontal planes and results from the rapid growth of the embryo. The growth rate at the sides of the embryonic disc fails to keep pace with the rate of growth in the long axis as the embryo increases rapidly in length. Folding at the cranial and caudal ends and sides of the embryo occurs simultaneously. Concurrently, there is relative constriction at the junction of the embryo and umbilical vesicle.

Folding of Embryo in the Median Plane
Folding of the ends of the embryo produces head and tail folds that result in the cranial and caudal regions moving ventrally as the embryo elongates cranially and caudally (see Fig. 5.1 A 2 to D 2 ).
Head Fold
At the beginning of the fourth week, the neural folds in the cranial region form the primordium of the brain (see Fig. 5.1 A 2 and B 2 ). Initially, the developing brain projects dorsally into the amniotic cavity, the fluid-filled cavity inside the amnion (the innermost membrane around the embryo). The amniotic cavity contains amniotic fluid and the embryo. Later, the developing forebrain grows cranially beyond the oropharyngeal membrane and overhangs the developing heart ( Fig. 5.2 B and C ). At the same time, the septum transversum , primordial heart, pericardial coelom, and oropharyngeal membrane move onto the ventral surface of the embryo. During folding, part of the endoderm of the umbilical vesicle is incorporated into the embryo as the foregut (primordium of pharynx, esophagus, and lower respiratory system) (see Fig. 5.2 C , and also see Chapter 11 ). The foregut lies between the forebrain and primordial heart, and the oropharyngeal membrane separates the foregut from the stomodeum , the primordial mouth ( Fig. 5.3 B , and see Fig. 5.2 C ).


After folding of the head, the septum transversum lies caudal to the heart, where it subsequently develops into the central tendon of the diaphragm , the partition between the abdominal and thoracic cavities (see Fig. 5.3 B , and also see Chapter 8 ). The head fold also affects the arrangement of the embryonic coelom (primordium of the body cavity). Before folding, the coelom consists of a flattened, horseshoe-shaped cavity (see Fig. 5.1 A 1 ). After folding, the pericardial coelom lies ventral to the heart and cranial to the septum transversum (see Fig. 5.2 B and C ). At this stage, the intraembryonic coelom communicates widely on each side with the extraembryonic coelom (see Figs. 5.1 A 3 and 5.3 A and B ).
Tail Fold
Folding of the caudal end of the embryo results primarily from growth of the distal part of the neural tube, the primordium of the spinal cord ( Fig. 5.4 A and B ). As the embryo grows, the caudal eminence (tail region) projects over the cloacal membrane , the future site of the anus (see Figs. 5.3 A and 5.4 B ). During folding, part of the endodermal germ layer is incorporated into the embryo as the hindgut , the descending colon and rectum (see Fig. 5.4 B ).

The terminal part of the hindgut soon dilates slightly to form the cloaca , the rudiment of the urinary bladder and rectum (see Fig. 5.4 B , and also see Chapters 11 and 12 ). Before folding, the primitive streak lies cranial to the cloacal membrane (see Fig. 5.4 A ); after folding, it lies caudal to it (see Fig. 5.4 B ). The connecting stalk (primordium of the umbilical cord) is now attached to the ventral surface of the embryo (see Fig. 5.4 A ), and the allantois, or the diverticulum of the umbilical vesicle, is partially incorporated into the embryo (see Fig. 5.4 A and B ).
Folding of Embryo in the Horizontal Plane
Folding of the sides of the developing embryo produces right and left lateral folds (see Fig. 5.1 A 3 to D 3 ). Lateral folding is produced by the rapidly growing spinal cord and somites. The primordia of the ventrolateral abdominal wall fold toward the median plane, rolling the edges of the embryonic disc ventrally and forming a roughly cylindric embryo (see Fig. 5.6 A ). As the abdominal wall forms, part of the endoderm germ layer is incorporated into the embryo as the midgut , the primordium of the small intestine (see Fig. 5.1 C 2 , and also see Chapter 11 ).
Initially, there is a wide connection between the midgut and umbilical vesicle (see Fig. 5.1 A 2 ); however, after lateral folding, the connection is reduced, forming an omphaloenteric duct (see Fig. 5.1 C 2 ). The region of attachment of the amnion to the ventral surface of the embryo is also reduced to a relatively narrow umbilical region (see Fig. 5.1 D 2 and D 3 ). As the umbilical cord forms from the connecting stalk (see Fig. 5.1 B 2 and D 2 ), ventral fusion of the lateral folds reduces the region of communication between the intraembryonic and extraembryonic coelomic cavities to a narrow communication (see Fig. 5.1 C 2 ). As the amniotic cavity expands and obliterates most of the extraembryonic coelom, the amnion forms the epithelial covering of the umbilical cord (see Fig. 5.1 D 2 ).
Germ Layer Derivatives
The three germ layers (ectoderm, mesoderm, and endoderm) formed during gastrulation ( Fig. 5.5 ) give rise to the primordia of all tissues and organs. The specificity of the germ layers, however, is not rigidly fixed. The cells of each germ layer divide, migrate, aggregate, and differentiate in patterns as they form the various organ systems. The main germ layer derivatives are as follows (see Fig. 5.5 ):
- •
Ectoderm gives rise to the central nervous system; peripheral nervous system; sensory epithelia of the eyes, ears, and nose; epidermis and its appendages (hair and nails); mammary glands; pituitary gland; subcutaneous glands; and enamel of the teeth. Neural crest cells , derived from neuroectoderm , the central region of early ectoderm, eventually give rise to or participate in the formation of many cells types and organs, including cells of the spinal cord, cranial nerves (V, VII, IX, and X), and autonomic ganglia; ensheathing cells of the peripheral nervous system; pigment cells of the dermis; muscles, connective tissues, and bones of pharyngeal arch origin; suprarenal medulla; and meninges (coverings) of the brain and spinal cord.
- •
Mesoderm gives rise to connective tissue, cartilage, bone, striated and smooth muscles, heart, blood, and lymphatic vessels; kidneys; ovaries; testes; genital ducts; serous membranes lining the body cavities (pericardial, pleural, and peritoneal membranes); spleen; and cortex of the suprarenal glands.
- •
Endoderm gives rise to the epithelial lining of the digestive and respiratory tracts; parenchyma (connective tissue framework) of the tonsils; thyroid and parathyroid glands; thymus, liver, and pancreas; epithelial lining of the urinary bladder and most of the urethra; and epithelial lining of the tympanic cavity, tympanic antrum, and pharyngotympanic tube (see Fig. 5.5 ).

Control of Embryonic Development
Embryonic development results from genetic plans in the chromosomes. Knowledge of the genes that control human development is increasing (see Chapter 21 ). Most information about developmental processes has come from studies of other organisms, especially zebrafish, chickens, and mice, because of the ethics associated with the use of human embryos for laboratory studies.
Most developmental processes depend on a precisely coordinated interaction of genetic and environmental factors. Several control mechanisms guide differentiation and ensure synchronized development, such as tissue interactions, regulated migration of cells and cell colonies, controlled proliferation, and programmed cell death (apoptosis) . Each system of the body has its own developmental pattern.
Embryonic development is essentially a process of growth and increasing complexity of structure and function. Growth is achieved by mitosis (somatic reproduction of cells) together with the production of extracellular matrices (surrounding substances), whereas complexity is achieved through morphogenesis and differentiation. The cells that make up the tissues of very early embryos are pluripotential (i.e., they have the capacity to affect more than one organ or tissue), which under different circumstances are able to follow more than one pathway of development. This broad developmental potential becomes progressively restricted as tissues acquire the specialized features necessary for increasing their sophistication of structure and function. Such restriction presumes that choices must be made to achieve tissue diversification.
Most evidence indicates that these choices are determined not as a consequence of cell lineage but rather in response to cues from immediate surroundings, including the adjacent tissues. As a result, the architectural precision and coordination that are often required for normal function of an organ appear to be achieved by the interaction of the organ’s constituent parts during development.
The interaction of tissues during development is a recurring theme in embryology. The interactions that lead to a change in the course of development of at least one of the interactants are called inductions . Numerous examples of such inductive interactions can be found; for example, during development of the eye , the optic vesicle induces the development of the lens from the surface ectoderm of the head. When the optic vesicle is absent, the eye fails to develop. Moreover, if the optic vesicle is removed and placed in association with surface ectoderm that is not usually involved in eye development, lens formation can be induced.
Clearly, then, development of a lens is dependent on the ectoderm acquiring an association with a second tissue. In the presence of neuroectoderm of the optic vesicle, the surface ectoderm of the head adopts a pathway of development that it would not otherwise have taken. In a similar fashion, many of the morphogenetic tissue movements that play such important roles in shaping the embryo also provide for the changing tissue associations that are fundamental to inductive tissue interactions .
The fact that one tissue can influence the developmental pathway adopted by another tissue presumes that a signal passes between the two interactants. Analysis of the molecular defects in mutant animal strains shows that abnormal tissue interactions occur during embryonic development, and studies of the development of animal embryos with targeted gene mutations have begun to reveal the molecular mechanisms of induction. The mechanism of signal transfer appears to vary with the specific tissues involved. In some cases, the signal appears to take the form of a diffusible molecule, such as a sonic hedgehog (Shh) , that passes from the inductor to the reacting tissue. In others, the message appears to be mediated through a nondiffusible extracellular matrix that is secreted by the inductor and with which the reacting tissue comes into contact. In still other cases, the signal appears to require that physical contacts occur between the inducing and responding tissues. Regardless of the mechanism of intercellular transfer involved, the signal is translated into an intracellular message that influences the genetic activity of the responding cells.
The signal can be relatively nonspecific in some interactions. The role of the natural inductor in a variety of interactions has been shown to be mimicked by a number of heterologous tissue sources and, in some instances, even by a variety of cell-free preparations. Studies suggest that the specificity of a given induction is a property of the reacting tissue rather than of the inductor. Inductions should not be thought of as isolated phenomena. Often they occur in a sequential fashion that results in the orderly development of a complex structure; for example, following induction of the lens by the optic vesicle, the lens induces the development of the cornea from the surface ectoderm and adjacent mesenchyme. This ensures the formation of component parts that are appropriate in size and relationship for the function of the organ. In other systems, there is evidence that the interactions between tissues are reciprocal. During development of the kidney, for instance, the ureteric bud (metanephric diverticulum) induces the formation of tubules in the metanephric mesoderm (see Chapter 12 ). This mesoderm, in turn, induces branching of the diverticulum that results in the development of the collecting tubules and calices of the kidney.
To be competent to respond to an inducing stimulus, the cells of the reacting system must express the appropriate receptor for the specific inducing-signal molecule, the components of the particular intracellular signal transduction pathway , and the transcription factors that will mediate the particular response. Experimental evidence suggests that the acquisition of competence by the responding tissue is often dependent on its previous interactions with other tissues. For example, the lens-forming response of the head ectoderm to the stimulus provided by the optic vesicle appears to be dependent on a previous association of the head ectoderm with the anterior neural plate.
The ability of the reacting system to respond to an inducing stimulus is not unlimited. Most inducible tissues appear to pass through a transient but more or less sharply delimited physiologic state in which they are competent to respond to an inductive signal from the neighboring tissue. Because this state of receptiveness is limited in time, a delay in the development of one or more components in an interacting system may lead to the failure of an inductive interaction. Regardless of the signal mechanism employed, inductive systems seem to have the common feature of close proximity between the interacting tissues. Experimental evidence has demonstrated that interactions may fail if the interactants are too widely separated. Consequently, inductive processes appear to be limited in space as well as by time. Because tissue induction plays such a fundamental role in ensuring the orderly formation of precise structures, failed interactions can be expected to have drastic developmental consequences (e.g., birth defects such as absence of the lens).
Highlights of Fourth to Eighth Weeks
The following descriptions summarize the main developmental events and changes in the external form of the embryo during the fourth to eighth weeks. The main criteria for estimating developmental stages in human embryos are listed in Table 5.1 .
Age (Days) | Figure Reference | Carnegie Stage | Number of Somites | Length (mm) * | Main External Characteristics † |
---|---|---|---|---|---|
20–21 | 9 | 1–3 | 1.5–3.0 | Flat embryonic disc. Deep neural groove and prominent neural folds. One to three pairs of somites present. Head fold evident. | |
22–23 | 5–6 | 10 | 4–12 | 1.0–3.5 | Embryo straight or slightly curved. Neural tube forming or formed opposite somites but widely open at rostral and caudal neuropores. First and second pairs of pharyngeal arches visible. |
24–25 | 5–7 | 11 | 13–20 | 2.5–4.5 | Embryo curved owing to head and tail folds. Rostral neuropore closing. Otic placodes present. Optic vesicles formed. |
26–27 | 5–8 | 12 | 21–29 | 3.0–5.0 | Upper limb buds appear. Rostral neuropore closed. Caudal neuropore closing. Three pairs of pharyngeal arches visible. Heart prominence distinct. Otic pits present. |
28–30 | 5–9 5–11 | 13 | 30–35 | 4.0–6.0 | Embryo has C -shaped curve. Caudal neuropore closed. Four pairs of pharyngeal arches visible. Lower limb buds appear. Otic vesicles present. Lens placodes distinct. Tail-like caudal eminence present. |
31–32 | 5–12 5–13 | 14 | ‡ | 5.0–7.0 | Lens pits and nasal pits visible. Optic cups present. |
33–36 | 15 | 7.0–9.0 | Hand plates formed; digital rays visible. Lens vesicles present. Nasal pits prominent. Cervical sinuses visible. | ||
37–40 | 16 | 8.0–11.0 | Foot plates formed. Pigment visible in retina. Auricular hillocks developing. | ||
41–43 | 5–14 | 17 | 11.0–14.0 | Digital rays clearly visible in hand plates. Auricular hillocks outline future auricle of external ear. Trunk beginning to straighten. Cerebral vesicles prominent. | |
44–46 | 18 | 13.0–17.0 | Digital rays clearly visible in foot plates. Elbow region visible. Eyelids forming. Notches between digital rays in the hands. Nipples visible. | ||
47–48 | 5–15 | 19 | 16.0–18.0 | Limbs extend ventrally. Trunk elongating and straightening. Midgut herniation prominent. | |
49–51 | 20 | 18.0–22.0 | Upper limbs longer and bent at elbows. Fingers distinct but webbed. Notches between digital rays in the feet. Scalp vascular plexus appears. | ||
52–53 | 5–16 | 21 | 22.0–24.0 | Hands and feet approach each other. Fingers are free and longer. Toes distinct but webbed. | |
54–55 | 22 | 23.0–28.0 | Toes free and longer. Eyelids and auricles of external ears more developed. | ||
56 | 23 | 27.0–31.0 | Head more rounded and shows human characteristics. External genitalia still have indistinct appearance. Distinct bulge still present in umbilical cord, caused by herniation of intestines. Caudal eminence (tail) has disappeared. |
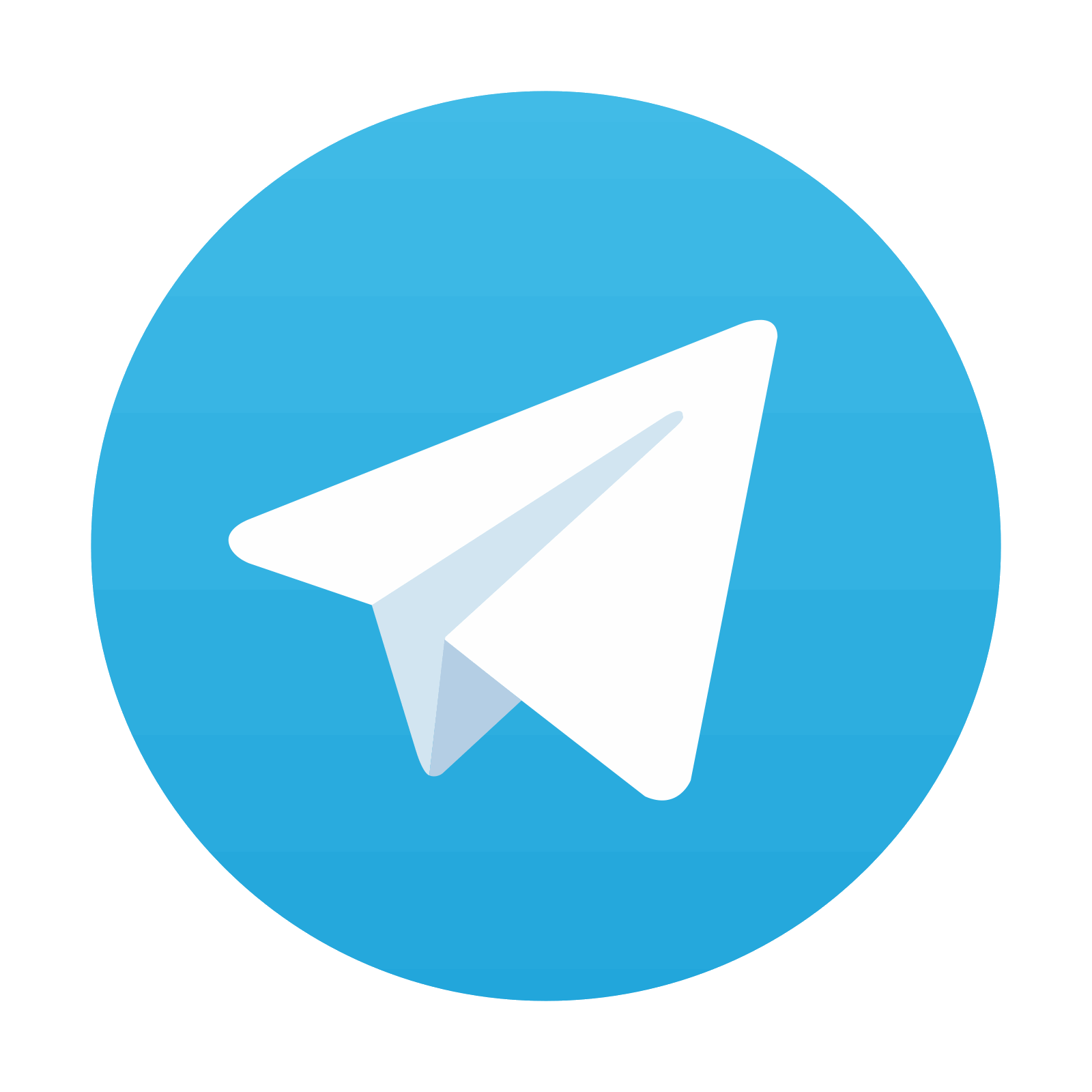
Stay updated, free articles. Join our Telegram channel
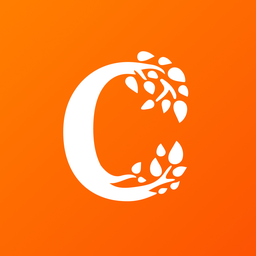
Full access? Get Clinical Tree
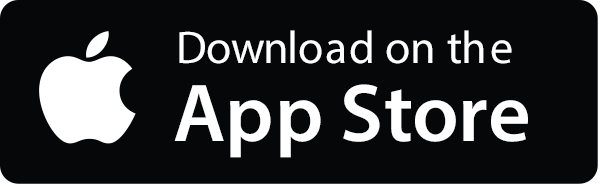
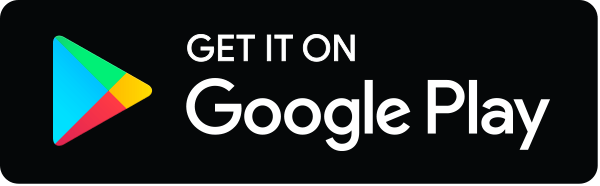
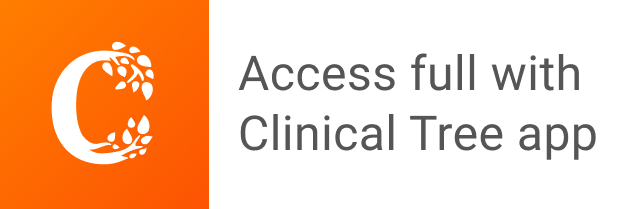