BRIEF HISTORY OF FETAL SURGERY
Sir William Liley (1963) completed the first successful fetal surgery, which was transuterine fetal intraperitoneal red blood cell transfusion. This was performed for erythroblastosis fetalis, which at the time, without intervention, was a lethal malady. The field rapidly developed, to a large extent fueled by the work of Dr. Michael Harrison, an early pioneer in fetal surgery. In 1982, one of the first organized conferences on fetal intervention was held. Dr. Harrison summarized conclusions of the inaugural meeting, which would later become the International Fetal Medicine and Surgery Society (IFMSS) (Harrison, 1982). One key statement noted that “All case material, regardless of outcome, should be reported to a fetal-treatment registry, so that the benefits and liabilities of fetal therapy can be established as soon as possible.” This ethos is as important today as it was three decades ago.
Fetal surgery has evolved during the past three decades from an innovative and ambitious concept to a regulated and leading field of medicine. Refinement of techniques for open surgeries, new technology, advances in minimally invasive image-guided percutaneous interventions, and development of feasible fetoscopic surgical procedures has fueled this evolution. In addition, protocols to control uterine contractions and preterm labor and to standardize care of neonates delivered preterm after surgery have been honed. Finally, specific fetal anesthesia considerations and intraoperative management algorithms contribute to improved fetal outcomes.
A fetal anomaly raises unique and complex issues for the pregnant woman and her family. The importance of a multidisciplinary team involved in the prenatal evaluation, surgical therapy, and postnatal care cannot be overemphasized. This team generally includes a maternal-fetal medicine specialist, pediatric surgeon, anesthesiologist skilled in maternal and fetal anesthesia, pediatric neurosurgeon, pediatric urologist, pediatric nephrologist, pediatric cardiologist, neonatologist, and bioethicist. The family should also have access to psychosocial support (Bliton, 2003).
CLOSED FETAL THERAPIES
Closed fetal therapies are procedures performed by inserting a needle or endoscopic trocar through the maternal abdominal and uterine wall without the need for hysterotomy. Most closed surgical procedures are performed under direct sonographic guidance and usually involve only one puncture, which typically measures 2.4 mm. This small puncture introduces a needle or trocar through which a shunt, balloon, or semirigid endoscope can be passed. Occasionally, closed fetal interventions are performed using a combination of sonographic and fetoscopic guidance.
In general, a policy should be adopted to administer fetal analgesics for any invasive procedures during which the fetus might experience pain. This is certainly true for 18- to 20-week or older gestations. Intramuscular or intravenous agents are delivered under sonographic or endoscopic guidance using an 22-gauge needle. We usually give vecuronium (0.2 mg/kg), atropine (20 μg/kg), and fentanyl (15 μg/kg) using estimated fetal weight to immobilize the fetus and to suppress the fetal stress response, which is bradycardia. For the mother, instrument insertion is usually done under local anesthesia, which is injected along the anticipated track of the cannula or trocar and extends down to the myometrium. Supplemental intravenous sedation with remifentanil is also usually provided.
The placentas of monochorionic, diamnionic (MCDA) twins frequently share vascular anastomoses. These anastomoses may be arterio-venous, veno-arterial, arterio-arterial, or veno-venous, and multiple types are typically found in a given placenta. Arterio-arterial and veno-venous anastomoses are connections on the placental surface and have the potential for either unidirectional or bidirectional blood flow. Arterio-venous and veno-arterial anastomoses are unidirectional and form when a placental surface vessel from each twin penetrates into the placenta and connects within a common cotyledon. Unbalanced flow through this shared cotyledon underlies the pathophysiology of twin–twin transfusion syndrome (TTTS) (Fig. 16-1). For MCDA twins, TTTS is not uncommon, and the estimated incidence ranges from 9 to 15 percent (Allaf, 2014b; Kagan, 2007; Lewi, 2007; Simpson, 1998).
The imbalance in circulating blood volume that results from these anastomoses directs blood from a donor twin to its recipient twin. This leads to relative hypovolemia in the donor twin and hypervolemia in the recipient. One effect of this hypervolemia and hypovolemia is markedly differing urine output, which is reflected by hydramnios and oligohydramnios, respectively. In addition, compensatory cardiovascular responses eventually become maladaptive. The donor twin usually maintains normal cardiac function. However, hypervolemia in the recipient twin increases cardiac preload, which leads to right ventricular hypertrophy and eventually to hypertension and cardiomyopathy. The increased systemic pressure may also increase right ventricular afterload and diminish right heart output, which contributes to an acquired pulmonic stenosis and eventual fetal death (Simpson, 1998). Such right ventricular outflow obstruction is observed in close to 10 percent of all recipient twins. Recipient twins who demonstrate cardiac compromise generally have poorer survival rates than their donor cotwin. Cardiac-compromised recipient twins may also have lower survival rates compared with recipient twins with normal cardiac function.
Serious neurologic complications can also result from these vascular anastomoses and include cerebral palsy, microcephaly, porencephaly, and multicystic encephalomalacia. One theory is that ischemic necrosis leads to cavitary brain lesions. In the donor twin, ischemia may result from hypotension, anemia, or both. In the recipient, ischemia may develop from blood pressure instability and episodes of severe hypotension (Lopriore, 2011).
The mortality rate for untreated progressive TTTS approximates 90 percent (Society for Maternal-Fetal Medicine, 2013). Thus, the primary goal of intervention is to restore more equitable blood flow between the twins and to halt or reverse cardiac decompensation in either fetus. Secondary efforts aim to relieve the fluid volume inequities between TTTS twins.
Early identification of TTTS offers the best chance to improve fetal outcomes. For first-trimester diagnosis, the evidence supporting the value of nuchal translucency thickness or crown-rump length discordancy for predicting adverse obstetric outcomes such as TTTS is conflicting. Kagan and coworkers (2007) noted a positive correlation for these measures and later development of TTTS. In contrast, one multicenter study found that first-trimester discordancy in nuchal translucency and crown-rump length measurements did not predict adverse obstetric or neonatal outcomes (Allaf, 2014b). However, this same study group did find that early second-trimester sonographic examination showing abdominal circumference and estimated fetal weight discordance may be associated with an increased risk of subsequent adverse obstetric outcome (Allaf, 2014a).
Fetal echocardiography is recommended in all MCDA twins at 20 to 24 weeks’ gestation. This is because the risk of cardiac anomalies is increased ninefold in these twins compared with general-population singletons. The prevalence of congenital cardiac anomalies has been reported to be 2 percent in otherwise uncomplicated MCDA gestations. In cases of TTTS, the prevalence is 5 percent, and a greater prevalence is seen in recipient fetuses (Bahtiyar, 2007). Functional fetal echocardiography may be valuable to detect subtle cardiac signs that can guide intervention timing and the overall management strategy for TTTS.
Treatment options for TTTS include selective fetal reduction, amnioreduction, amnionic septostomy, and laser photocoagulation of the surface placental anastomoses (Moise, 2005; Saade, 1998; Senat, 2004). Of these, amnioreduction uses paracentesis to pierce the uterus and amniochorion and allow excess amnionic fluid to be removed. Associated risks with this technique are preterm premature rupture of the membranes (PPROM), infection, placental abruption, preterm delivery, and neurologic complications in survivors.
With amnionic septostomy, a fetoscope and associated laser are placed transuterine. The laser creates a sizable window in the intervening amnion wall that separated MCDA twins to allow fluid equalization between the sacs. With this procedure, survival rates of 18 to 83 percent for both twins and rates of 80 percent for at least one twin have been reported (Moise, 2005).
With laser photocoagulation, a fetoscope is introduced transuterine under sonographic guidance via a 9F to 12F ported vascular catheter. Once anastomoses are identified, a diode or neodymium-doped yttrium aluminum garnet (Nd:YAG) laser and a 400- or 600-micron fiber are introduced to ablate surface placental vessel anastomoses (Fig. 16-2). This procedure is most commonly performed under local anesthesia.
FIGURE 16-2
In this image, the recipient twin (left) lies within a hydramnios-expanded sac, while the donor twin (right) is “stuck” against the uterine wall. The fetoscope is seen crossing the uterine wall and is positioned inside the uterus, over the vascular equator, and between the two cord insertions.

Several randomized trials and reviews have examined neonatal outcomes with these procedures. First, in one trial, amnion septostomy and serial amnioreduction were compared, and fetal outcomes were similar between the groups (Moise, 2005). Another randomized trial compared amnioreduction with laser photocoagulation and found similar obstetric complications. However, laser therapy offered a significantly higher survival rate and fewer reported neurologic abnormalities in survivors (Senat, 2004). One subsequent Cochrane review also compared laser therapy and septostomy. These authors found no differences in the overall death rate or in the death of at least one fetus per pregnancy (Roberts, 2014). Moreover, treatment groups did not differ in the death rates of both twins in a given pregnancy. However, more children were alive without neurologic abnormality at age 6 years in the laser group compared with the amnioreduction group. Of children that were alive at 6 years with major neurologic abnormalities, the number from each treatment group was similar. Notably, death outcomes in this 2014 update differ from the previous 2008 Cochrane review. In the earlier review, rate improvements in perinatal death and death of both fetuses per pregnancy were found in the laser intervention arm (Roberts, 2008). Despite the lack of effect on the death rate, the authors of the newer review conclude that endoscopic laser coagulation of anastomotic vessels should continue to be considered in the treatment for all stages of TTTS due to improve neurodevelopmental outcomes (Roberts, 2014).
In an attempt to standardize nomenclature for this condition, Quintero and colleagues (1999) classified TTTS progression into five stages based on the degree of fetal compromise (Table 16-1). Few programs offer intervention for stage I. Most consider laser photocoagulation only for Quintero stage II or higher stages, or stage I with extenuating circumstances that include rapidly worsening hydramnios or signs of impending cardiac compromise. The gestational age beyond which laser photocoagulation should not be offered also varies between programs. Some centers do not offer this therapy after 24 weeks’ gestation, whereas others use thresholds of 25 or 26 weeks. There has recently been a call to consider offering laser therapy between 17 weeks’ and 26 weeks’ gestation based on data suggesting equivalent outcomes (Baud, 2013). This has however not been universally adopted, and further research is awaited.
Stage | Classification |
---|---|
I | Amnionic fluid volume is discordant: oligohydramnios with a maximum vertical pocket (MVP) ≤2 cm in one sac and hydramnios in other sac (MVP ≥8 cma). The bladder of the donor twin is visible and Doppler studies are normal |
II | Amnionic fluid volume is discordant. The bladder of the donor twin is not visible (during the length of examination, usually around 1 hour), but Doppler studies are not critically abnormal |
III | Criteria above plus Doppler studies are critically abnormal in either twin and are characterized as abnormal or reversed end-diastolic velocities in the umbilical artery, reverse flow in the ductus venosus, or pulsatile umbilical venous flow |
IV | Fetal ascites, pericardial or pleural effusion, scalp edema, or overt hydrops |
V | One or both babies are dead |
Different surgical techniques have been proposed for performing fetoscopic laser ablation. Initially, nonselective laser coagulation of placental vessels was performed, and any vessels that crossed the intertwin membrane, that is, along the membranous equator, were coagulated (De Lia, 1990). Alternatively, selective laser coagulation of placental vessels (SLCPV) identifies and coagulates only anastomoses that cross between the twins along the vascular equator (Ville, 1995). A variant SLCPV procedure coagulates the surface of the placenta to linearly connect ablated anastomotic sites and thereby create a physical separation of donor and recipient vascular territories (Chalouhi, 2011). This is known as solomonization or the Solomon technique. In a controlled trial, 274 gravidas with twins affected by TTTS were randomly assigned to traditional laser coagulation or to the Solomon technique. Laser coagulation of the entire vascular equator significantly reduced rates of twin anemia polycythemia sequence and of TTTS recurrence, but perinatal mortality and severe neonatal morbidity rates did not differ (Slaghekke, 2014). The superiority of the Solomon technique has also been reported in other studies (Ruano, 2013, 2014).
Of technical hurdles to laser photocoagulation, a completely anterior placenta limits where the fetoscope can be inserted and can thereby hinder straight-on viewing of vessels. Also, the semirigid fetoscope limits angling of the scope. Inadequate visualization of the vascular equator impedes satisfactory ablation of all anastomoses. In addition, as the angle of incidence between the fetoscope and the placental surface becomes more obtuse, laser energy becomes less effective. To overcome these obstacles, a midline laparotomy may be performed to allow the uterus to be exteriorized and the fetoscope to enter the posterior uterine wall (Deprest, 1998). Alternatively, an endoscope with a 70-degree lens can be coupled with intracannula laser deployment (Quintero, 2010). Our team also uses a laparoscopic-assisted approach. With this, a traditionally positioned laparoscope visually guides fetoscope insertion from the woman’s dorsal flank and into the posterior uterus. Once inserted, the fetoscope can view anastomoses more directly (Shamshirsaz, 2015).
Similar to TTTS, fetofetal transfusion syndrome (FFTS) has been described in both dichorionic and monochorionic triplet gestations. FFTS may develop in 5 percent of dichorionic triplets, and in approximately 8 percent of monochorionic triplets (Blumenfeld, 2015). The perinatal demise in cases of early-onset FFTS in monochorionic-triamnionic or dichorionic-triamnionic triplet pregnancies is substantial. Laser photocoagulation of communicating anastomoses appears to improve neonatal survival rates but is technically more challenging and is potentially associated with lower success rates.
Lower urinary tract obstruction (LUTO) comprises a heterogeneous group of anatomic anomalies of the bladder neck. The two most common are posterior urethral valves, which are obstructing membranes in the proximal male urethra, and urethral atresia in either male or female fetuses (Gunn, 1995). Most urinary tract blockages are minor and not associated with significant morbidity (Wu, 2009). Complete obstruction, however, is associated with major consequences such as bladder dilation and hydroureteronephrosis. If complete urethral obstruction develops before glomeruli are fully formed, renal dysplasia results (Kitagawa, 1999). Subsequent anhydramnios can lead to pulmonary hypoplasia and skeletal deformities. Almost 45 percent of untreated cases with severe obstruction end in neonatal death (Makayama, 1986). LUTO should be confirmed postnatally to ensure an accurate diagnosis.
LUTO is commonly found during the sonographic fetal anatomic survey at 18 to 20 weeks’ gestation. Typical findings include a dilated posterior urethra (keyhole sign), an enlarged bladder (megacystis), and unilateral or bilateral hydronephrosis with or without a cystic appearance of the renal parenchymal (cystic kidney disease). It has been diagnosed as early as 12 weeks’ gestation. But, data are few, and the first-trimester diagnosis of LUTO requires further research (Byon, 2013).
A detailed sonographic examination is performed to exclude coexisting anomalies. For some cases, amnioinfusion may create an acoustic window to improve fetal anatomy viewing. Fetal gender should be confirmed, as a female fetus significantly increases the likelihood of complex malformations such as urethral atresia, persistent cloaca, or megacystis microcolon intestinal hypoperistalsis syndrome. Assessment of the fetal karyotyping is also offered to exclude aneuploidy. In our center, to ensure adequate tissue for karyotyping, we perform placental biopsy or fetal blood sampling rather than amniocentesis or, in cases of anhydramnios, fetal vesicocentesis. In addition, examination of fetal urine samples obtained by vesicocentesis may provide some information regarding the degree of renal impairment. Reference ranges for normal fetal urine have been developed, and prognostic values have been proposed for specimens collected between 18 and 22 weeks’ gestation (Table 16-2) (Muller, 1996). However, the potential for predicting postnatal renal function using sodium, calcium, and β2-microglobulin indices was questioned in a 2007 systematic review of 23 studies (Morris, 2007).
Good Prognosis | Poor Prognosis | |
---|---|---|
Sodium | <90 mmol/L | >100 mmol/L |
Chloride | <80 mmol/L | >90 mmol/L |
Osmolality | <180 mOsm/L | >200 mOsm/L |
Calcium | <7 mmol/L | >8 mmol/L |
Total protein | <20 mg/dL | >40 mg/dL |
β2-Microglobulin | <6 mg/L | >10 mg/L |
More recently a review of 72 cases of LUTO concluded that fetal urinalysis before 23 weeks’ gestation allowed distinction between three groups. These are: (1) fetuses with normal urine biochemistry for which fetal therapy should be discussed; (2) fetuses with abnormal urine biochemistry results that portend poor renal outcome, for which the benefit of fetal therapy is likely to be compromised; and (3) fetuses with urodigestive fistula (Abdennadher, 2014). In our center, we obtain urine for biochemical testing as part of the initial evaluation that incorporates fetal karyotype, echocardiography, and full sonographic anatomic scan. If the initial biochemistry is favorable, vesicoamnionic shunting may be considered without a second specimen. If the results are unfavorable, a second specimen is obtained 48 hours later and tested. If still unfavorable, shunting is not recommended. In the future, proteomic and metabolomic evaluation of fetal urine and/or blood may offer better prognostic information, but this is not currently standard of care (Klein, 2013).
Antenatal intervention most commonly entails serial percutaneous fetal bladder drainage or placement of a double-pigtail vesicoamnionic shunt to relieve obstruction and thus prevent some or all sequelae. Using intravenous sedation and local anesthesia, shunt placement usually begins with amnioinfusion of warmed saline or Ringer lactate solution into the amnionic cavity under sonographic guidance. This creates a space to aid visualization of the fetus and also provides sufficient room around the fetus for correct deployment of the shunt’s external pigtail loop. With sonographic guidance, the maternal abdomen and then fetal abdomen and bladder are punctured using a needle or trocar, through which the double-pigtail catheter is passed (Fig. 16-3). The proximal end is positioned and curls within the fetal bladder, whereas the distal end is deployed in the amnionic space (Fig. 16-4). Complications with vesicoamnionic shunt are catheter displacement, catheter occlusion from thrombus material, procedure-related placental abruption, PPROM, preterm labor, and preterm birth. However, these are less common than open fetal surgery or fetoscopic procedures.
The Percutaneous Vesicoamniotic Shunting Versus Conservative Management for Lower Urinary Tract Obstruction (PLUTO) trial was performed to assess the effectiveness of vesicoamnionic shunting. Unfortunately, because of poor recruitment, this trial was stopped after only 31 patients were enrolled. Despite this small sample size, data showed that shunted fetuses had better survival rates than nonshunted fetuses. No conclusions could be made regarding the effects of vesicoamnionic shunting on long-term renal function (Morris, 2013).
As an alternative procedure, some investigators report ablation of posterior urethral valves using fetal cystoscopy combined with either mechanical or laser disruption of the posterior urethral valves (Ruano, 2015). Because of current equipment limitations and the potential for fistula formation following laser fulguration, this approach should be regarded as experimental (Sananes, 2015).
Fetuses with pleural effusion or fluid-filled, space-occupying chest lesions may be candidates for thoracoamnionic shunt placement. Physiologically, these two chest conditions increase hydrostatic pressure within the fetal thorax. Depending on severity, this may result in pulmonary hypoplasia or compression of the fetal heart that leads to cardiac decompensation and nonimmune hydrops.
This fluid collection in the pleural space develops in 1 in 10,000 to 15,000 pregnancies. Pleural effusions can present as an isolated or primary finding or as a secondary complication of an associated fetal malformation or karyotypic abnormality. Most isolated pleural effusions reflect a chylothorax that results from abnormal drainage of lymph directly into the pleural space. With secondary pleural effusions, comorbid conditions include fetal aneuploidy, cardiac anomalies, congenital infection, lymphatic anomaly, anemia, congenital cystic adenomatoid malformation, bronchopulmonary sequestration, or congenital diaphragmatic hernia and have been reported in 25 to 75 percent of cases (Rustico, 2007). The two anomalies most commonly associated with pleural effusions are congenital diaphragmatic hernia and trisomy 21. Other chest pathology that must be considered includes tracheoesophageal fistula, extralobar pulmonary sequestration, and congenital pulmonary lymphangiectasis.
Thus, careful diagnostic evaluation and patient selection are crucial. For this, a detailed sonographic fetal anatomic survey and echocardiography are essential, and fetal karyotyping is recommended (Achiron, 1995). Maternal blood type, antibody status, Kleihauer-Betke testing, and virology testing that screens for toxoplasmosis, rubella, and cytomegalovirus, herpes simplex virus, and parvovirus B19 infections are also recommended to exclude other potential causes of fetal hydrops. The National Institute for Health and Clinical Excellence (NICE) guideline (2006) on this topic states that invasive fetal therapy for fetal hydrothorax should be restricted to fetuses with isolated effusions resulting in hydrops. Some experienced clinicians have suggested intervention should be considered for cases of fetal hydrops with the pleural effusion as the likely etiology or for cases without hydrops but with an isolated pleural effusion that occupies more than 50 percent of the thoracic cavity, causes a shift in mediastinum, rapidly increases in size, or leads to hydramnios (Yinon, 2010). That said, we do not believe that intervention is helpful in nonhydropic fetuses with a unilateral pleural effusion.
Thoracocentesis under sonographic guidance is generally the first approach and removes as much pleural fluid as possible. The fluid is assessed to identify chylothorax, and a high lymphocyte count, usually >80 percent, confirms this diagnosis. After fluid drainage, the degree of fetal lung reexpansion is assessed sonographically, and the lungs are simultaneously evaluated to exclude underlying structural abnormalities. Fetuses in whom the effusion rapidly reaccumulates may benefit from thoracoamnionic shunt placement.
Importantly, pleural fluid must be differentiated from a massive pericardial effusion, which in some cases can look similar. The size and shape of the lungs can help differentiate the two. Namely, massive pericardial effusion will compress and flatten the lungs against the posterior chest wall and cause them to appear very small. However, with a pleural effusion, the lungs are usually seen alongside the heart. The most common cause of massive pericardial effusion is right ventricular aneurysm (Hara, 2007). Attempting to drain either of these can be counterproductive. In most cases, unless cardiac function is compromised, massive pericardial effusion from an aneurysm can be expectantly managed and will usually resolve.
The technique for shunt placement for pleural effusion mirrors that for cystic masses, described next. For nonhydropic fetuses with isolated pleural effusion, in utero intervention is associated with a survival rate of 60 to 85 percent. For hydropic fetuses with pleural effusions, rates are 50 to 60 percent (Deurloo, 2007; Knox, 2006; Yinon, 2010). For nonhydropic fetuses, shunt placement in cases of isolated bilateral pleural effusion currently offers, at most, a small increase in survival rates (Knox, 2006).
Another congenital abnormality that may benefit from thoracoamnionic shunting is congenital cystic adenomatoid malformation (CCAM), also known as congenital pulmonary adenomatoid malformation (CPAM). A CCAM is a bronchopulmonary malformation usually identified sonographically as an intrapulmonary mass commonly localized to one lung lobe. The differential diagnosis includes mediastinal teratoma, congenital diaphragmatic hernia, and bronchopulmonary sequestration. The incidence of CCAM ranges between 1 in 25,000 to 1 in 35,000 live births (Laberge, 2001). These malformations lack normal alveoli but instead show excessive proliferation and cystic dilatation of terminal respiratory bronchioles. CCAMs are classified based on the sonographic size of the cyst(s) (Stocker, 1977). Macrocystic (Stocker type I) lesions contain at least one cyst measuring >5 mm, whereas microcystic (Stocker type III) lesions appear echogenic and lack visible cysts. Type II lesions are mixed.
During evaluation, fetal pulmonary lesions should be evaluated using color Doppler to differentiate CCAM from bronchopulmonary sequestration. This latter lesion is associated with systemic blood supply from an anomalous aortic vessel. Magnetic resonance (MR) imaging is also useful for imaging fetal chest masses. This modality can help to distinguish CCAMs from other intrathoracic lesions, to localize the lesion to a specific lobe, and to visualize the compressed normal lung (Hubbard, 1999). Fetal echocardiography assists in excluding cardiac anomalies and in evaluating cardiac function in fetuses with evolving or fulminant hydrops. Karyotyping is not indicated for CCAMs unless associated anomalies are present. Most fetuses with antenatally detected CCAMs have a good outcome. Microcystic CCAMs tend to regress spontaneously after a mass growth peak at 26 to 28 weeks’ gestation (MacGillivray, 1993). Those CCAMs that remain small, do not contain cysts >1 cm, and do not demonstrate a mass effect within the chest at the time of a 32- to 33-week sonographic examination are unlikely to cause respiratory symptoms after birth. These can be expectantly managed. However, macrocystic lesions generally do not regress, as fluid accumulates in the cysts. Hydrops is the single best predictor of fetal death with CCAMs. Thus, with macrocysts, sonographic surveillance is performed one or two times weekly in fetuses to seek signs of hydrops and monitor changes in CCAM volume.
The CCAM volume ratio (CVR), a sonographically derived ratio, helps identify fetuses at risk for developing hydrops and who therefore require more frequent monitoring. The CCAM volume is measured by using the formula for an ellipse (length × height × width × 0.52). A CVR is obtained by dividing the CCAM volume (mL) by the head circumference (cm) to correct for differences in fetal gestational age. A CVR ≤1.6 correlates with a 94-percent survival rate and a less than 3-percent risk of developing hydrops (Crombleholme, 2002). Investigations are ongoing to determine whether cardiovascular function assessment, such as calculation of combined cardiac output, may also help identify fetuses at risk for hydrops (Mahle, 2000).
Choosing an appropriate candidate with a macrocystic CCAM for thoracoamnionic shunt placement remains challenging. CCAMs with a large, dominant cyst have responded favorably to thoracoamnionic shunt placement. Shunt placement in hydropic fetuses with macrocystic CCAMs can promote hydrops reversal (Adzick, 1998; Grethel, 2007; Smith, 2005). Thus, shunting should be considered for hydropic fetuses with macrocystic lung lesions. This is especially true for fetuses with very large lung lesions that enlarge rapidly or are associated with fetal cardiac compromise or hydramnios (Schrey, 2012). At present, the benefit and safety of thoracoamnionic shunt in fetuses with macrocystic lesions and no hydrops is unclear. In fetuses with a chest mass and hydrops, our group has noted that unless the hydrops is associated with cardiac decompensation, intervention may also be unnecessary (Cass, 2012). We administer betamethasone to women whose fetus has a large, high-risk CCAM. Some data suggest that between two and four courses of betamethasone may help improve survival rates in these high-risk cases. Although the mechanism is unclear, steroids may induce CCAM regression or bolster fetal tolerance of associated hydrops (Derderian, 2015).
As part of the selection process prior to shunting, initial macrocyst needle drainage allows the percent decline in mass volume to be measured. This correlates with the lung volume gained if the cyst were chronically drained. Prior to needle insertion, color-flow Doppler is used to identify an avascular zone between the fetal chest wall and the dominant macrocyst. Once positioned at the thoracic wall, a 22-gauge needle is sonographically guided into the center of the dominant macrocyst. Observation of cyst decompression helps determine the optimal position for a shunt should the macrocyst reappear. Sonographic chest reevaluation is done 2 to 3 days after this aspiration to look for reaccumulation (Adzick, 1998).
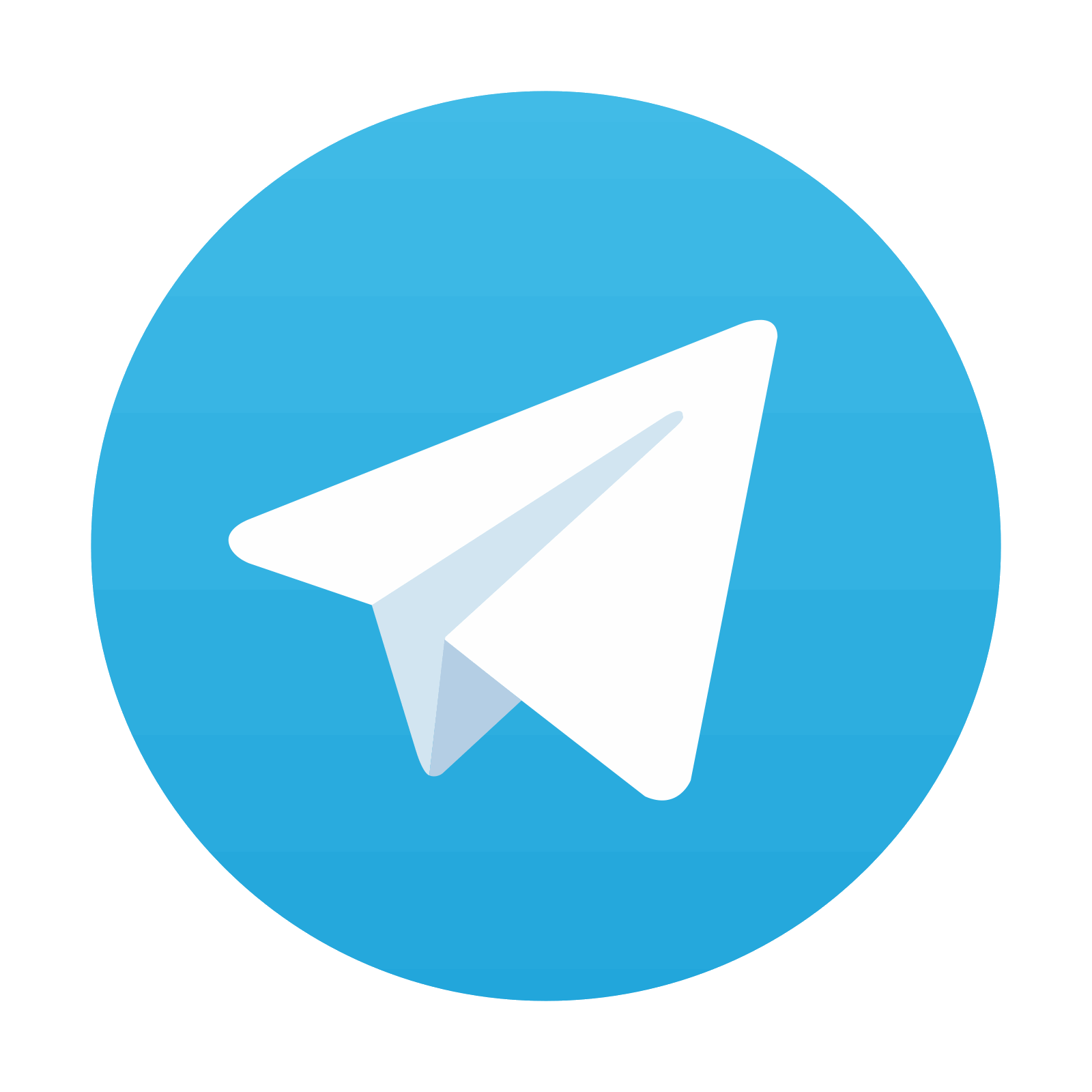
Stay updated, free articles. Join our Telegram channel
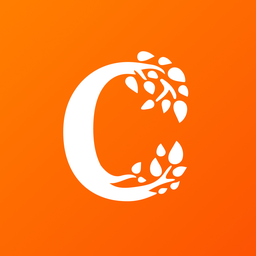
Full access? Get Clinical Tree
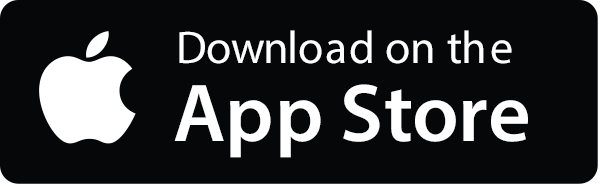
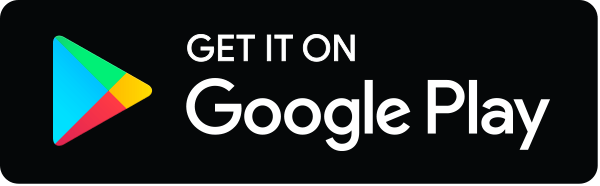