Endocrine adaptation to extrauterine life
The endocrine system plays a vital role in fetal development and the transition from intrauterine to extrauterine life. The endocrine pathways are not static, but rather complex and constantly adapting to regulation by hormones, nutrients, cytokines, and growth factors.
Growth hormone (GH), prolactin, and the insulin-like growth factors (IGFs)
Random GH levels in the fetus are high (~30 to 35 ng/mL) at midgestation. Values decline toward term but generally exceed 10 ng/mL within the first week of life (Figure 20-1). Thus random GH levels are useful for the diagnosis of GH deficiency before a week of age.
The decline of GH is associated with a rise in serum insulin-like growth factor 1 (IGF-1) and insulin-like growth factor binding protein 3 (IGF BP-3) levels, reflecting an increase in hepatic sensitivity to GH. Nevertheless, IGF-1 levels are low in the first year of life, making them unreliable for assessment of GH production or action. IGF BP-3 levels are more useful for diagnosis of GH deficiency in early life. Still, both IGF BP-3 and in particular IGF-I are modulated by nutritional status and systemic health. Consequently, the levels of IGF-1 and (to a lesser degree) IGF BP-3 are often low in malnourished, ill infants even if their ability to produce GH is normal.
Prolactin levels can be as high as 400 ng/mL within the first few weeks of life in normal infants. Levels are higher in patients with hypothalamic disease and lower in infants with primary pituitary disease or those treated with dopamine.
Thyroid function
In the fetus, T4 and TSH levels rise progressively after midgestation; T3 levels increase later after 30 weeks (Figure 20-2).
In full-term infants, the rapid shift in ambient temperature (from 37°C to 23°C) stimulates a striking rise in TSH, which peaks at ~70 μU/mL within 45 to 60 minutes after birth. This is followed by increases in both T4 and T3, which attain levels (T4, 13 to 22 μg/dL; T3, 250 to 400 ng/dL) seen in hyperthyroidism in older children and adults. T4 and T3 decline progressively after the first week of life but remain slightly higher than those in older children throughout the first 6 to 8 months. TSH levels decline after the first week but can be as high as 7.5 μU/mL before 6 months of age.
In preterm infants, the basal T4, T3, and TSH levels are low; this reflects immaturity of the hypothalamic-pituitary-thyroid axis. Differentiation of this hypothyroxinemia of prematurity from central hypothyroidism or the sick euthyroid syndrome can be difficult.
Findings that suggest true central (hypopituitary) hypothyroidism include midfacial hypoplasia, midline cleft lip or palate, nystagmus (seen with optic nerve hypoplasia), midline defects in CNS development such as holoprosencephaly, third ventricular hemorrhage, microphallus, and abnormalities in the levels of other pituitary hormones (eg, low GH, cortisol, or testosterone; high or low prolactin; or diabetes insipidus).
Patients with presumed or proven central hypothyroidism should be treated with thyroxine; otherwise normal infants with isolated hypothyroxinemia of prematurity or sick euthyroid syndrome should not be treated with thyroxine unless the serum T4 and T3 levels fail to rise as expected with age or with recovery from illness.
Certain children are at high risk for thyroid dysfunction: these include infants with Down syndrome, Turner syndrome, DiGeorge syndrome, and Prader-Willi syndrome. These children require thyroid function testing on a routine basis: prior to discharge from the nursery and every 6 months thereafter (or more frequently depending on clinical status).
Adrenal function
There are progressive decreases in serum 17-hydroxyprogesterone (17OHP), dehydroepiandrosterone (DHEA), and DHEA-sulfate (DHEAS) levels in the fetus during late gestation and an increase in serum cortisol near term (Figure 20-3). These reflect maturation of adrenal cortical enzymes (21-hydroxylase and 3β-hydroxysteroid dehydrogenase) and increases in adrenal cortisol production.
Thus, babies born before 30 to 32 weeks of gestation have relative immaturity of corticoadrenal function and are at risk for adrenal insufficiency (see Chapter 10).
Measurement of serum ACTH and cortisol (prior to administration of hydrocortisone) is useful for diagnosis of adrenal insufficiency. In some cases, an ACTH stimulation test can be used to assess corticoadrenal function.
Note that 17OHP levels are particularly high in preterm infants, complicating the evaluation for congenital adrenal hyperplasia (21OHase deficiency); clinical assessment and repeat blood studies can determine if the high levels of 17OHP are physiologic (ie, due to prematurity) or pathologic.
Babies with suspected or proven adrenal insufficiency (from any cause) must receive supplemental hydrocortisone during severe febrile illnesses or with intractable vomiting, trauma, or surgery. Parenteral hydrocortisone should be administered if the child is acutely ill and/or is unable to tolerate PO medications.
Gonadal function
In full-term infants, the serum testosterone and dihydrotestosterone (DHT) levels rise into the low normal adult range within the first week of life, decline, and then rise again between days 20 and 60 of life (Figure 20-4). Thus the diagnosis of gonadal insufficiency can be made in week 1 and, if the child is otherwise well, in the second month of life.
After month 2, the testosterone levels are very low and diagnostically useless until the onset of puberty.
Estradiol levels are very high in the fetus, causing breast development and, in females, withdrawal bleeding after birth. The levels of estradiol fall below the limits of detectability soon after birth and remain very low until puberty.
Effects of prematurity on endocrine function
There is progressive maturation of endocrine function during fetal and neonatal development (Table 20-1). Preterm infants are, therefore, at high risk for endocrine and metabolic dysfunction.
Glucose intolerance
Premature infants are predisposed to glucose intolerance because β-cell mass and insulin production increase dramatically during the late fetal and neonatal periods.
Insulin secretion is commonly excessive in infants of diabetic mothers (IDM) and in infants born small for gestational age (SGA), who may have a transient (weeks to months) form of hyperinsulinism associated with hypoglycemia. The hypoglycemia in IDM can persist for as long as 5 to 7 days; more prolonged hypoglycemia should prompt further investigation. The hyperinsulinism in SGA infants generally responds to diazoxide.
Cortisol deficiency
This is common in preterm infants because of immaturity of corticoadrenal function and iatrogenic suppression.
Infants in the NICU are commonly treated with tapering doses of hydrocortisone, following the need for stress dosing early in their hospital course for hypotension or renal insufficiency. The exogenous hormone suppresses function of the hypothalamic-pituitary-adrenal axis.
Thyroxine deficiency
The hypothyroxinemia of prematurity may be exacerbated by the stress of illness, malnutrition, and certain medications (dopamine, glucocorticoids, and antiepileptics) that suppress TSH secretion and inhibit the peripheral conversion of T4 to T3.
Children with hypothyroxinemia of prematurity or with low levels of T4, T3, and TSH resulting from illness or medications should, in general, not be treated with thyroxine. However, thyroxine treatment is indicated in children with true central hypothyroidism.
Following withdrawal of medications and recovery from illness, the rebound rise in serum TSH precedes the rise in T4 or T3; this can simulate primary hypothyroidism but generally requires no intervention unless high levels of TSH persist.
Growth hormone deficiency
Acute and chronic illness and malnutrition are associated with hepatic resistance to GH action and consequent low levels of IGF-1.
Recovery from illness and nutritional rehabilitation is accompanied by increases in serum IGF1 and IGF BP-3 and an increase in rate of linear growth.
The gonadal axis is suppressed by stress or malnutrition; thus testosterone levels are difficult or impossible to interpret in acutely or chronically ill children who are poorly nourished. In such cases, gonadotropin (LH and FSH) levels are low.
Prolactin is under tonic inhibition by the hypothalamus. Consequently, high levels of prolactin may serve as a marker of hypothalamic dysfunction. On the other hand, primary pituitary disorders may be associated with hypoprolactinemia.
CNS disorders associated with endocrinopathies of the high-risk infant
Pituitary hormone deficiencies can present anytime beginning in the newborn period.
It is critical to evaluate all pituitary hormones when there is concern for any pituitary dysfunction.
Specific reference values will vary depending on the age of the infant and laboratory assay used.
Since the hypothalamus and pituitary are midline structures, any midline defects such as cleft lip, cleft palate, or microphallus should raise suspicion for possible hypothalamic or pituitary dysfunction.
Table 20-2 lists some of the common acquired and congenital CNS disorders that can disrupt the hypothalamicpituitary axis and result in deficits in one or more pituitary hormones.
A pediatric ophthalmologist should evaluate any child with pituitary dysfunction for optic nerve hypoplasia. This can vary in clinical severity from nystagmus to blindness and may be associated with one or more hypothalamic-pituitary deficiencies. Conversely, any child found to have optic nerve hypoplasia should be referred immediately for evaluation to a pediatric endocrinologist.
A brain MRI with fine cuts through the pituitary region will determine the size and structural configuration of the hypothalamic-pituitary region. Imaging may identify defects of other CNS midline structures known to be associated with pituitary dysfunction, such as the optic nerves, corpus callosum, and septum pellucidum.
Absence of the septum pellucidum and thinning or absence of the corpus callosum are commonly observed in septooptic dysplasia, a syndrome characterized by optic nerve hypoplasia, midline brain abnormalities, and hypopituitarism, which may include central diabetes insipidus. Though most cases of septooptic dysplasia are due to sporadic mutations, defects in the genes, HESX1, SOX2, and OTX2, have been described in familial cases.
Disorders of glucose metabolism
In utero, the infant receives nutrition via a continuous transplacental infusion of glucose, amino acids, and essential fatty acids. Within 2 hours of birth, blood glucose concentrations in healthy term newborns tend to fall. To maintain euglycemia, the infant’s insulin levels decline while counterregulatory hormones, such as epinephrine and glucagon, stimulate breakdown of hepatic glycogen stores to provide sufficient energy for up to 4 to 6 hours. This process is followed by gluconeogenesis, a process largely controlled by cortisol.
Hypoglycemia
Hypoglycemia in infants and children, if unrecognized or untreated, can lead to seizures, developmental delay, permanent brain damage, and death.
Definition
Neonatal hypoglycemia is best defined as the blood glucose concentration at which intervention should be initiated to avoid morbidity, particularly neurologic effects.
The precise definition of neonatal hypoglycemia has been controversial. Beyond the first day of life, this can be defined as blood glucose less than 50 mg/dL. However, many clinicians argue that blood sugars in full-term infants should be maintained above 65 mg/dL.
Incidence: Very common, especially in premature infants and infants of diabetic mothers.
Pathophysiology: Generally, the etiology of hypoglycemia can be classified into four main categories.
Inadequate glucose supply
Inadequate glycogen stores
Impaired glucose production (ie, glycogenolysis or glucone-ogenesis)
Increased glucose utilization
The differential diagnosis of neonatal hypoglycemia is very broad, ranging from a delay in adaptation to extrauterine life to more permanent endocrine or metabolic disorders.
Risk factors: Prematurity, IUGR, LGA, stress, sepsis, IDM, certain maternal drugs, polycythemia, hypothermia, inadequate caloric intake, conditions associated with hyperinsulinism.
Clinical presentation
Symptoms of hypoglycemia are nonspecific and may include jitteriness, tremors, hypotonia, irritability, lethargy or stupor, apnea, bradycardia, cyanosis, tachypnea, poor feeding, weak cry, hypothermia, or seizures.
Blood glucose should be checked when any of these symptoms are present.
Management of hypoglycemia
Immediate workup needs to be initiated upon confirmation of hypoglycemia.
Table 20-3 and Figure 20-5 list the critical labs to be obtained at the time of hypoglycemia (<50 mg/dL) and their interpretation. Urine ketones can be checked at the time of, or soon after, the hypoglycemic event.
Following critical lab collection, and prior to correcting hypoglycemia, a glucagon stimulation test should be performed: Glucagon 0.5 to 1 mg is given intramuscularly. Finger stick blood glucose measurements should be checked prior to and at 10, 20, and 30 minutes postinjection; serum GH and cortisol are obtained 60 and 90 minutes postinjection. No oral or IV glucose should be administered during the 30-minute period unless the child becomes symptomatic.
If hypoglycemia is not corrected at 30 minutes with glucagon, or if the infant is at any point symptomatic, then hypoglycemia should be corrected by feeding or IV bolus of D10W or D25W.
Additional labs (Table 20-3) to evaluate metabolic disorders can be obtained when the infant is euglycemic.
A positive response to glucagon (Figure 20-5), defined as a rise in blood glucose concentration exceeding 30 mg/dL, strongly suggests hyperinsulinism. Additional findings suggesting hyperinsulinism include a high glucose infusion requirement (>12 mg/kg/min), a normal or elevated insulin level at the time of hypoglycemia, the absence of urinary ketones (this may also be seen in defects of fatty acid oxidation), and low levels of IGF-binding protein 1 (IGF-BP1).
Hyperinsulinism can be acquired or congenital, transient or permanent. Acquired causes of hyperinsulinism include the transient hyperinsulinism in IDM and infants born SGA. Congenital causes of hyperinsulinism can be transient or persistent. Persistent causes are usually associated with defects in genes that regulate insulin secretion. Activating mutations in glutamate dehydrogenase are associated with elevated ammonia and lactate at time of hypoglycemia. Hyperinsulinism can occur in up to 50% of patients with Beckwith-Wiedemann syndrome (see Table 20-4, which can be transient or persistent.
Hyperinsulinism in SGA infants and in genetic disorders is initially treated with diazoxide. High drug doses (>10 mg/kg/d) are associated with fluid retention and require concurrent use of a diuretic. Hypertrichosis and coarse facial changes can occur with chronic use of diazoxide. This will resolve with decreased dosing or discontinuation of the drug. Diazoxideresistant hyperinsulinism may require a continuous infusion of glucagon or octreotide. In the most severe cases, partial pancreatectomy may be required; such procedures are performed in a limited number of medical centers around the world.
Other causes of hypoglycemia should be sought if there is a negative glucagon response. A normal or low cortisol (<15 μg/dL) or GH (<10 ng/mL) level at the time of hypoglycemia suggests the possibility of pituitary and adrenal dysfunction. GH deficiency can be isolated or associated with other pituitary hormone deficiencies. Primary adrenal insufficiency, with high ACTH and low cortisol levels, is exceedingly rare in the neonate; imaging of bilateral adrenals should be performed to rule out bilateral adrenal hemorrhage, which may manifest with flank masses. Other causes of neonatal hypoglycemia (Figure 20-5) include increased glucose utilization without hyperinsulinism (eg, sepsis, heart failure, polycythemia, hypothermia). Severe hepatic dysfunction can impair both glycogenolysis and gluconeogenesis.
Convalescent and follow-up care
All parents of infants with hyperinsulinism should be discharged home with glucagon emergency kits and glucometers, and must demonstrate understanding of proper administration and use.
Follow-up with a pediatric endocrinologist should be arranged.
Hyperglycemia
Definition
Normal fasting blood sugars range fall below 100 mg/dL; random or postprandial sugars fall below 140 mg/dL.
Plasma glucose levels >150 mg/dL at any gestational age or postnatal age is considered hyperglycemic.
Diabetes mellitus is defined as fasting blood glucose >125 mg/dL fasting and/or postprandial or random glucose ≥200 mg/dL.
Incidence: Very common in extreme prematurity or the setting of stress and sepsis. Neonatal diabetes occurs in ~1 in 500,000 births.
Pathophysiology
Both sepsis and the stress response to critical illness can cause hyperglycemia, particularly in preterm infants. This is due in part to the rise of epinephrine and cortisol in response to stress. In sepsis, there is also a reduction in peripheral utilization of glucose. The concomitant rise in cortisol reduces peripheral glucose utilization, causing insulin resistance, while simultaneous suppression of insulin secretion by catecholamines reduces the insulin response to glucose. This is compounded by prematurity, a risk factor for hyperglycemia due to low β-cell mass.
Pancreatitis is a cause of persistent hyperglycemia; levels of amylase and lipase are diagnostic. Marked elevations of triglycerides (>500 mg/dL) may induce pancreatitis.
Neonatal diabetes is associated with an absolute and permanent deficiency of insulin and C-peptide. Unlike type 1 diabetes mellitus, which is an autoimmune disorder, it is usually caused by a single gene mutation and presents before 6 months of age. Depending on the associated gene mutation, it may be characterized by other syndromic physical features. Table 20-5 lists the common gene defects causing neonatal diabetes. Three of the genes, also linked with neonatal hypoglycemia, encode proteins that play a critical role in the normal function of the ATP-sensitive potassium channel that regulates insulin secretion. Neonatal diabetes can be permanent or transient.
Risk factors: ELBW, stress, sepsis, TPN use and/or lipid infusion, use of caffeine or corticosteroids, seizures.
Clinical presentation
Infants are typically asymptomatic of hyperglycemia.
Signs of dehydration from osmotic diuresis, including tachycardia, tachypnea, depressed anterior fontanelle, poor skin turgor, dry mucous membranes.
Signs of sepsis.
Management of hyperglycemia
The first step in evaluating neonatal hyperglycemia is to rule out iatrogenic causes, such as a high glucose infusion rates and use of glucocorticoids.
Once iatrogenic causes are eliminated, the following critical labs should be obtained at the time of hyperglycemia: insulin, C-peptide, amylase and lipase, and lipid panel (Table 20-6). These may help determine the cause of the hyperglycemia (Figure 20-6).
Initial treatment typically requires intravenous insulin infusion until sufficient subcutaneous fat stores develop.
Subsequently, the neonate can be transitioned to an insulin pump with continuous subcutaneous delivery of insulin, using diluted insulin if necessary. This allows for administration of very small doses of insulin, otherwise difficult to administer via a syringe or pen device.
Convalescent and follow-up care
Long-term management requires regular visits with a pediatric endocrinologist. Responsiveness to insulin therapy can be judged in the outpatient setting with daily home glucose monitoring, measurements of HbA1c every 3 months, and monitoring for appropriate weight gain, growth, and development.
Disorders of thyroid function
Thyroxine synthesis: The hypothalamus secretes thyrotropinreleasing hormone (TRH), which stimulates the pituitary to secrete thyroid-stimulating hormone (TSH). TSH then binds to receptors on thyroid follicular cells to promote thyroid growth, iodine uptake, and synthesis of thyroxine (T4) and triiodothyronine (T3). The majority of T4 and T3 are bound to transport proteins: 75% to 80% to thyroxine-binding globulin (TBG), 10% to 15% to transthyretin or albumin. Less than 1% of T4 and T3 are unbound and circulate in the periphery as free T4 and T3. Deiodinases in the pituitary and peripheral tissues regulate tissue-specific conversion of T4 to T3, the more bioactive thyroid hormone. Up to 80% of T3 is synthesized outside of the thyroid gland.
Thyroid function: Thyroid hormone has a wide range of key functions. In the fetus, it is essential for early brain development and skeletal maturation. T3 increases basic metabolic rate via increased oxygen consumption and heat production. This is important immediately following birth as the infant adapts to the cold extrauterine environment. Thyroid hormone has other metabolic effects on heart, liver, kidneys, skin, and skeletal muscle.
Evaluation of thyroid function requires assessment of pituitary TSH secretion and circulating free T4 (Figure 20-7). T3 levels are also useful, particularly in stressed infants with low T4 and normal or low TSH. Specific reference values of these hormones vary depending on laboratory assay used as well as gestational age and weight (see Tables 20-7 and 20-8).
Hypothyroidism
Hypothyroidism can result from defects in thyroid development, thyroid hormone production (primary hypothyroidism), pituitary TSH secretion (secondary or central hypothyroidism), or thyroid hormone action (Figure 20-7, Table 20-9).
Primary congenital hypothyroidism is characterized by marked elevations of TSH and low free T4.
In the developed world, the most common cause of permanent primary congenital hypothyroidism is thyroid dysgenesis due to either ectopic thyroid gland or thyroid hypoplasia/aplasia. The second most common cause is thyroid dyshormonogenesis, an umbrella term referring to a defect in thyroid hormone production.
Signs and symptoms of congenital hypothyroidism may include widened fontanelle, macroglossia, umbilical hernia, jaundice, bradycardia, poor feeding, and a weak cry.
However, the great majority of infants with congenital hypothyroidism are asymptomatic. Therefore, close attention to state newborn screening results is essential.
Treatment (levothyroxine, 10 to 15 μg/kg/d) must be initiated as soon as possible, as delay in therapy may lead to worse outcome. Infants treated with levothyroxine before 10 days of age are more likely to have normal or near-normal neurodevelopment.
Secondary or central hypothyroidism is characterized by a low normal or low TSH and low free T4.
Cases of central hypothyroidism result from hypothalamic-pituitary dysfunction and should prompt investigation of overall pituitary function (see Section I.C).
Treatment, as in primary hypothyroidism, consists of levothyroxine supplementation.
Central hypothyroidism must be distinguished from the more common hypothyroxinemia of prematurity and the sick euthyroid syndrome. The former has low levels of T4 and T3 and normal or low TSH; if the child is born before 30 weeks of gestation, the T3 levels may be markedly reduced. The latter has normal or low T4, marked reduction in T3, and normal TSH. Reverse T3 levels are elevated. Some drugs, particularly dopamine, glucocorticoids, and antiepileptics, will also suppress TSH and free T4.
Mutations in the thyroid hormone receptor present with high T4 and T3 levels and normal or high TSH. Since the heart may retain its sensitivity to thyroid hormone, patients are commonly treated with β-blockers to reduce heart rate and limit the risk of arrhythmias. Patients with mutations in the gene for monocarboxylase transporter 8 (MCT8), which mediates T3 transport into the brain, have severe hypotonia and cognitive impairment, low or normal values of TSH and free T4 (Figure 20-7), and striking elevations of T3. Novel treatments for this condition are currently in clinical trials; to be successful, any therapy will have to be initiated soon after (or even before) birth in order to prevent irreversible brain damage.
A deficiency of TBG is characterized by a low total T4 but with normal TSH and free T4 levels. It is more prevalent in males as it is X-linked. Since free T4 is normal, these patients are euthyroid and do not require treatment.
Hyperthyroidism
While rare, infants born to women with Graves disease are at risk of developing neonatal Graves disease due to transplacental crossing of maternal thyrotrophic receptor antibodies (TRAbs).
These antibodies can have inhibitory, stimulatory, or neutral effects on the neonate’s thyroid. If stimulatory, they can cause transient (2 to 6 months) neonatal hyperthyroidism (Table 20-10 with suppressed TSH and elevated free T4 and total T3 levels.
An infant born to a woman with a history of Graves disease is at risk of neonatal Graves disease even if the mother has had definitive treatment and is currently euthyroid. This is because definitive therapies do not block TRAbs synthesis.
Thioamides (methimazole or propylthiouracil) used for treatment of maternal Graves disease also cross the placenta and may either suppress the stimulatory effects of TRAbs if present, or directly block fetal thyroid hormone synthesis, causing transient hypothyroidism. Babies born to mothers treated with thioamides should be monitored closely for the first 2 weeks of life; the drugs are cleared from the infant’s circulation by 5 to 7 days of life, so the emergence of clinical and biochemical hyperthyroidism may be delayed.
Permanent neonatal hyperthyroidism, while rare, may be caused by activating mutations of the TSH receptor. This can occur as an isolated mutation or as part of McCune-Albright syndrome. In these cases, neither the infant nor the mother has thyroid antibodies.
Treatment of neonates with hyperthyroidism is aimed at decreasing thyroid hormone synthesis with methimazole. Iodides may be used to block thyroid hormone release. β-Blockers are used to treat tachycardia and hypertension. In some cases, the infant may require therapy with glucocorticoids.
Disorders of thyroid function
Calcium regulation
Calcium levels are under tight regulation and maintained in a narrow range to allow for proper cellular and neuronal functioning (Table 20-11).
Half of the circulating calcium is bound to proteins, mainly albumin, while the other half circulates as free and metabolically active ionized calcium. Total serum calcium should be corrected in cases of hypoalbuminemia.
Ionized calcium, however, is not affected by albumin levels, but is modulated by blood pH. Alkalosis decreases ionized calcium by increasing binding to albumin; acidosis increases ionized calcium by decreasing binding to albumin.
Ionized calcium is under tight control via the actions parathyroid hormone (PTH) and 1,25 dihydroxy vitamin D (1,25[OH]2D).
PTH acts on two principal target organs: bone and kidney. PTH stimulates bone resorption to release stored calcium and phosphorus. In the kidney, PTH causes tubular reabsorption of calcium in the distal tubule while inhibiting reabsorption of phosphate in the proximal tubule. PTH also stimulates 1α-hydroxylase in the kidney to produce 1,25[OH]2D, which then acts on the intestinal mucosa to increase calcium and phosphate absorption. The net effect of PTH is, therefore, to increase serum calcium while decreasing serum phosphorus.
PTH secretion is regulated by negative feedback by calcium via the calcium-sensing receptor (CaR) and is induced by hyperphosphatemia and impaired by abnormalities in magnesium: hypomagnesemia impairs PTH secretion, while hypermagnesemia reduces PTH secretion and action. Therefore, the first step in evaluating either hypo- or hypercalcemia is to measure and, if necessary, normalize serum magnesium levels.
Hypercalcemia
Though hyperparathyroidism due to a CaR mutation is the most common cause of persistent neonatal hypercalcemia, other causes (Figure 20-8) should first be excluded. These include medications (particularly thiazides, vitamin D), adrenal insufficiency, hyperthyroidism (due to increased bone resorption), subcutaneous fat necrosis, and malignancy. Tertiary hyperparathyroidism only occurs in the setting of renal insufficiency. In the newborn period, tuberculosis is the most common granulomatous disease and known to cause hypercalcemia; this is due to increased 1,25[OH]2D production in the lymphoid tissue and pulmonary macrophages.
Symptoms of severe hypercalcemia include lethargy, coma, weakness, hypertension, arrhythmia (leading to asystole), shortened QT interval, nephrocalcinosis, vomiting, and constipation. Patients with mild hypercalcemia may be asymptomatic.
Evaluation of hypercalcemia involves measurement of PTH, phosphorus, creatinine, 1,25[OH]2D, and 25-OH vitamin D, and urine calcium, phosphorus, and creatinine. See Tables 20-11 and 20-12 for reference values of calcium and phosphorous in neonates. PTH is low in patients with vitamin D intoxication, Williams syndrome, and hypophosphatasia, in which alkaline phosphatase levels are markedly diminished for age.
PTH is inappropriately normal or elevated in cases of familial hypocalciuric hypercalcemia (FHH), an autosomal dominant disorder. The heterozygous form is associated with mild asymptomatic hypercalcemia, mild hypophosphatemia, and hypocalciuria. The homozygous form presents with severe hypercalcemia and more striking elevations of PTH, which can resemble a picture of primary hyperparathyroidism. FHH is caused by loss-of-function mutations in the CaR in the parathyroid gland and kidney. These mutations shift the set point to inhibit PTH release to the right, meaning a higher concentration of calcium is required to shut off PTH secretion. In the kidney, the CaR regulates urinary calcium excretion; as in the parathyroid, a higher calcium concentration is required to allow for calcium excretion. The heterozygous form of FHH is benign and does not require treatment.
Treatment of symptomatic hypercalcemia: Start with saline hydration. Loop diuretics (ie, furosemide) promote calcium excretion. Therapies for severe hypercalcemia may include calcitonin or bisphosphonates. Chronic therapy is aimed at the underlying cause of hypercalcemia. Parathyroidectomy is often required for treatment of homozygous FHH.
Hypocalcemia
Hypocalcemia in the neonate is broadly classified as either early or late hypocalcemia (Figure 20-9). Early hypocalcemia occurs in the first 4 days of life and can be the result of prematurity, birth asphyxia, maternal diabetes, or RBC transfusion. Blood transfusions may contain citrate as an anticoagulant, which binds to calcium and reduces levels of ionized calcium. Late hypocalcemia occurs or persists beyond the fourth day of life.
Symptoms of hypocalcemia include tetany, seizures, cataracts, calcifications, prolonged QT interval, and arrhythmias.
PTH and 1,25[OH]2D play key roles in preventing hypocalcemia. Therefore, PTH secretion should be optimized by correcting hypo- or hypermagnesemia. Primary hypoparathyroidism can be familial, postsurgical, or idiopathic. Biochemical features include an inappropriately low or undetectable PTH with high serum phosphorous, high urine calcium, and low urine phosphorus. Inherited causes of hypoparathyroidism include heterozygous (autosomal dominant) activating mutations in the CaR, which presents with hypocalcemia and hypercalciuria, and X-linked or autosomal recessive mutations of the PTH gene. Other causes include DiGeorge syndrome (22q11.2 microdeletion), iron-deposition diseases (ie, transfusion-dependent blood disorders, hemochro-matosis), and severe magnesium depletion, which can temporarily paralyze PTH secretion.
Pseudohypoparathyroidism is an inherited disorder in which the target organs are unresponsive to PTH. Therefore, biochemically, it mimics primary hypoparathyroidism, but is associated with significantly high PTH levels. One form of pseudohypoparathyroidism is Albright hereditary osteodystrophy, manifested by short stature, round face, short digits and metatarsals, subcutaneous ossification, and decreased cognition. This is caused by a loss of function of the gene encoding the stimulatory G-protein α subunit (GNASα) affecting target organ response to PTH as well as other hormones, such as TSH, LH, FSH, and GHRH. These patients rarely initially present with hypocalcemia; they are more likely to be diagnosed first with primary hypothyroidism and later found to have resistance to PTH.
Evaluation: Figure 20-9 shows an algorithm for the various causes of hypocalcemia based on critical lab studies.
Rickets: The combination of hypocalcemia, elevated PTH, and reduction in vitamin D levels should prompt evaluation for rickets. Table 20-13presents the biochemical findings of rickets when classified as calcipenic versus phosphopenic. Calcipenic rickets is associated with low serum phosphorus and high PTH. Further classification depends on serum levels of 25-OH vitamin D and 1,25[OH]2D. Nutritional rickets is caused by low dietary calcium or combined deficiency of dietary calcium and vitamin D. Vitamin D–dependent rickets type 1 mimics vitamin D deficiency, but serum 1,25[OH]2D is reduced preferentially because of a genetic mutation in renal 1α hydroxylase. Vitamin D–resistant rickets, also known as vitamin D–dependent rickets type 2, is caused by a mutation of the gene encoding the vitamin D receptor. This leads to vitamin D resistance and high levels of both 25-OH vitamin D and 1,25[OH]2D. Phosphopenic rickets is associated with low serum phosphorous and normal PTH. This can be secondary to nutritional deficiencies or renal loss of phosphate or inherited mutations of phosphate regulating genes. X-linked hypophos-phatemic rickets is an inherited disorder caused by mutation of the phosphate-regulating endopeptidase on the X chromosome (PHEX) gene. This results in renal phosphate wasting. Hereditary hypophosphatemic rickets with hypercalciuria has an autosomal recessive inheritance and is distinguished by an elevated 1,25[OH]2D and increased urinary calcium excretion.
Treatment: The acute treatment of hypocalcemia is to administer intravenous calcium followed by oral calcium and vitamin D supplementation. Chronic therapy includes oral calcium and vitamin D supplementation.
In hypoparathyroid states, the serum calcium levels should be maintained in the low normal (or slightly below normal) range; close monitoring of calcium levels is essential to prevent overtreatment. Follow-up renal ultrasounds are recommended to detect early signs of nephrocalcinosis.
Disorders of thyroid function
Water metabolism
Antidiuretic hormone (ADH, also called arginine vasopressin) is synthesized in the hypothalamus and secreted from the posterior pituitary. Along with thirst, it regulates serum osmolality through renal water reabsorption. Only a 3% to 4% increase in osmolality (versus a 10% decrease in blood volume) is required to trigger ADH secretion.
Aldosterone, a mineralocorticoid, is produced by and released from the adrenal gland under the control of the renin-angiotensin-aldosterone system (RAAS). Its function is to excrete potassium in exchange for sodium and water.
Renin is released by the kidneys secondary to sympathetic nerve activation, intravascular volume depletion, renal artery hypotension, or decreased renal sodium delivery. Renin activates angiotensin-converting enzyme (ACE) to form angiotensin II, which acts on the adrenal cortex to release aldosterone and stimulates the release of ADH from the posterior pituitary.
Hyponatremia
In the initial evaluation, three main causes of pseudohyponatremia should first be excluded: hyperlipidemia, hyperproteinemia, and hyperglycemia. In these cases, the increase in plasma lipids, protein, or glucose causes water to exit cells. This results in a falsely low serum sodium concentration though the measured serum osmolality is normal.
Figure 20-10 differentiates hyponatremia according to volume status: hypovolemia, euvolemia, and hypervolemia.
If the infant is clinically hypovolemic, then the next step is to measure urine sodium excretion. (Note: use of diuretics renders the urine sodium concentration uninterpretable.) Low urine sodium in the context of low serum sodium and osmolality indicates extrarenal causes of hyponatremia; these include intestinal losses (vomiting, diar-rhea) or salt and water loss from the skin (as in cystic fibrosis). High urine sodium in the context of hyponatremia and hypoosmolality points to mineralocorticoid deficiency or renal sodium loss from loop diuretics, osmotic diuresis (eg, high dextrose or hypertonic fluids), or intrinsic renal tubular disease. Infants who experienced CNS manipulation may develop cerebral salt wasting, which is thought to result from impaired sympathetic input to the adrenals, causing impaired renin and aldosterone release and the production of natriuretic factors by the brain. Urine sodium levels generally exceed 150 mmol.
Euvolemic hyponatremia can be the result of diuretics, hypothyroidism, glucocorticoid deficiency, or SIADH. Untreated long-standing hypothyroidism leads to decreased renal perfusion and impairs free water clearance.
Syndrome of inappropriate antidiuretic hormone (SIADH) requires four main criteria: decreased serum osmolality, inappropriate urine concentration, clinical euvolemia, and increased urine sodium excretion. Causes of SIADH include drugs (eg, anesthetics), CNS disease (eg, trauma, infection), and ectopic production (including pneumonia).
Hypervolemic hyponatremia has four main causes: renal disease, cirrhosis, congestive heart failure, and hypoalbuminemia. Most patients have obvious edema.
Signs and symptoms: Clinically symptomatic hyponatremia usually occurs at levels less than 120 mEq/mL and can include seizures, coma, or respiratory failure.
Treatment of hyponatremia is determined by severity and duration of hyponatremia. When symptomatic, the serum sodium needs to be raised rapidly with hypertonic saline to levels at which symptoms abate. However, if the hyponatremia is chronic and the infant is asymptomatic, then the serum sodium must be corrected slowly to prevent an osmotic demyelination syndrome, formerly known as central pontine myelinolysis. The safest therapy is restricting free water intake with close monitoring as the serum sodium corrects over several days. The goal is to raise serum sodium not more than 0.5 mmol/h.
Hypernatremia
Figure 20-11 reviews the workup for hypernatremia.
Like hyponatremia, diuretics can also cause hypernatremia and should be excluded before further evaluation.
In evaluating hypernatremia, measurements of urine output and serum and urine osmolality are particularly important. An elevated urine output with elevated serum osmolality and relatively low urine osmolality is consistent with the inability to concentrate urine, suggesting a diagnosis of diabetes insipidus (DI).
DI has two forms: central in which the posterior pituitary does not secrete sufficient ADH and nephrogenic, in which vasopressin receptors in the kidneys do not respond to ADH.
Central DI can result from defects in CNS development (including septooptic dysplasia), mutations in genes encoding vasopressin or its associated protein neurophysin, genetic disorders of thirst, trauma, or hypothalamic surgery. This form of DI is treated with ADH replacement using a vasopressin continuous infusion acutely and then DDAVP administered orally or by subcutaneous injection.
Congenital nephrogenic DI is inherited as either an X-linked recessive mutation of the vasopressin receptor or an autosomal recessive mutation of the renal aquaporin water channels. This form usually presents in the first week of life with vomiting, failure to thrive, and polyuria. Treatment is aimed at reducing urine volume with a thiazide diuretic and low sodium diet.
Acquired nephrogenic DI in the neonatal period is most commonly due to intrinsic renal disease (eg, polycystic kidneys, renal infarcts), hypokalemia, or hypercalcemia. Maintenance of normal potassium and calcium concentrations is required for appropriate function of renal aquaporins. Treatment of acquired nephrogenic DI involves correcting electrolyte imbalances or the underlying renal disease.
Infants with hypernatremia, oliguria, or anuria, elevated serum osmolality, and concentrated urine likely have hypertonic dehydration. Additional causes of hypertonic dehydration include insensible water loss, GI tract losses (vomiting, diarrhea), or incorrect formula preparation. Treatment involves correcting the precipitating problem.
Secondary hyperaldosteronism is a complication of any disorder associated with intravascular volume depletion or diminished renal blood flow. Most of these disorders are associated with normal or low serum sodium concentrations.
Hypernatremic conditions in the newborn period associated with increases in mineralocorticoid activity include two rare conditions.
Glucocorticoid suppressible hyperaldosteronism (GSH), in which the promoter for 11β-hydroxylase (11βOH) is fused to the coding region for aldosterone synthase, resulting in hyperaldosteronism, low renin, and hypertension. This is suppressed by treatment with glucocorticoids, which lower ACTH levels and reduces stimulation of the 11βOH promoter.
Apparent mineralocorticoid excess is associated with sodium and water retention and hypertension despite low renin and aldosterone levels. This condition is caused by a mutation in 11β-hydroxysteroid-dehydrogenase type 2 (11β-HSD2), which normally converts cortisol to inactive cortisone. The resulting excess of cortisol binds to mineralocorticoid receptors in the renal tubule, causing an apparent mineralocorticoid excess.
Disorders of thyroid function
Embryologic development
Between 4 and 6 weeks of gestation, the urogenital ridge gives rise to the bipotential gonad, which then differentiates into either testes or ovaries depending on the presence of specific genes. The most important of these is the SRY gene (testes determining factor), located on the Y chromosome. If absent, the bipotential gonad undergoes the process of ovarian differentiation.
The epididymis, vas deferens, ejaculatory duct, and seminal vesicle stem from the Wolffian ducts. The uterus, fallopian tubes, and upper third of the vagina stem from the mullerian ducts.
In infants with functional testes, Sertoli cells secrete antimullerian hormone (AMH), also known as mullerian-inhibiting hormone (MIH), which promotes regression of the mullerian ducts around 7 weeks’ gestation.
Leydig cells produce testosterone, which helps stabilize the Wolffian ducts around 8 to 9 weeks of gestation. The conversion of testosterone to dihydrotestosterone by the enzyme 5α-reductase is responsible for development and differentiation of the male external genitalia.
Defects of testosterone synthesis or action lead to Wolffian duct regression and external female genitalia differentiation.
Evaluation of disorders of sexual differentiation
The findings on physical examination suggesting a DSD are listed in Table 20-14 These include micropenis, hypospadias, undescended testes, clitoromegaly, and/or posterior labial fusion. It is important to closely examine the phallic size, symmetry of the external genitalia, appearance of scrotum or labiae, and presence and location of palpable gonads.
The anogenital ratio is measured as the distance between the anus and the posterior fourchette of the vagina divided by the distance between the anus and the base of the phallic structure. An anogenital ratio greater than 0.5 suggests 46,XX DSD with virilization and posterior labial fusion.
The examination should determine whether findings are consistent with gestational age and evaluate for additional dysmorphic features to help identify syndromes associated with DSD (Table 20-15).
If gonads are palpable and symmetric (Figure 20-12), then the infant may have 46,XY DSD. If the gonads are palpable, but asymmetric, then the child may have mixed gonadal dysgenesis. Lastly, if the gonads are not palpable, then the infant may have either 46,XY DSD or 46,XX DSD (undervirilized male or virilized female).
46,XY DSD with palpable gonads
For 46,XY DSD with palpable gonads (Figure 20-13), imaging is obtained in addition to laboratory evaluation of testosterone (T), DHT, and AMH.
If no uterus or other mullerian structures are detected, then SRY and AMH production were intact to cause regression of mullerian ducts.
A high testosterone to dihydrotestosterone ratio suggests 5α-reductase deficiency, an autosomal recessive disorder associated with a small phallus, perineal hypospadias, and blind vaginal pouch.
High levels of both testosterone and DHT suggest a diagnosis of androgen insensitivity syndrome (AIS) caused by mutation of the androgen receptor. This X-linked condition impairs testosterone action. In complete AIS, the phenotype is female with a blind vaginal pouch and absent uterus. Abdominal gonads need to be removed prior to age 18 years to prevent gonadoblastoma.
Low levels of both testosterone and DHT suggest an androgen synthesis defect (Table 20-16), including some forms of CAH (congenital lipoid adrenal hyperplasia, 3β-hydroxysteroid dehydrogenase deficiency, 17OHase deficiency, and p450 oxidoreductase deficiency) and the Smith-Lemli-Opitz syndrome. The latter is an autosomal recessive disorder with multiple malformations, including mental retardation, facial dysmorphisms (upturned nose, micrognathia, cleft palate), failure to thrive, and urogenital abnormalities (46,XY DSD, hypospadias, cryptorchidism). The mutation in Smith-Lemli-Opitzresults in decreased activity of 7-dehydrocholesterol reductase, which is the last step in cholesterol synthesis. 7-Dehydrocholesterol concentrations are, therefore, markedly elevated.
46,XY DSD with mullerian structures on imaging have low levels of AMH caused by mutations of the AMH gene. This condition is called persistent mullerian syndrome.
Lastly, ovotesticular DSD (previously termed true hermaphroditism) occurs with mutation of genes responsible for sexual differentiation. The gonads contain both ovarian tissue with follicles and testicular tissue with seminiferous tubules. This can form an ovotestis, or in some cases an ovary on one side and testis on the other. The karyotype is 46,XX in ~2/3 of cases. Levels of T, DHT, and AMH are variable depending on the degree of testicular function.
46,XX DSD or 46,XY DSD with nonpalpable gonads
When the gonads are not palpable (Figure 20-14), imaging is necessary to assess presence or absence of abdominal gonads; however, it may be difficult to distinguish between a testis and ovary.
Cryptorchidism, or undescended testes, is the most common disorder of sexual differentiation but does not present with ambiguous genitalia. Spontaneous testicular descent may occur in infancy, but referral to urology should be made if testes have not descended by 6 months of age as early intervention with orchiopexy may prevent possible testicular neoplasm and preserve testicular function and fertility.
If gonads are absent and the infant has 46,XY karyotype, the child most likely as a form of gonadal dysgenesis. There are bilateral streak gonads and a female phenotype without clinical features of Turner syndrome (45,XO).
Patients with 46,XX DSD (Figure 20-15) and a uterus have a virilizing disorder. Maternal virilization is attributed to transplacental passage to the fetus of androgens from an exogenous source or an androgen secreting tumor.
Placental aromatase deficiency is a rare autosomal recessive disorder in which there is progressive maternal virilization during pregnancy (hirsutism, clitoral hypertrophy, acne, frontal balding) due to decreased aromatase activity. Aromatase plays an important role in conversion of androgens to estrogens. Therefore, the levels of T, DHT, and androstenedione are elevated with low estrogen concentrations.
Congenital adrenal hyperplasia
Any virilized infant with 46,XX DSD requires evaluation for congenital adrenal hyperplasia (CAH).
CAH is caused by mutations in the genes encoding steroidogenic enzymes involved in cortisol biosynthesis. These genes include 3β-hydroxysteroid dehydrogenase type 2 (3β-HSD2), 21-hydroxylase (CYPE21), and 11β-hydroxylase (CYP11B1).
Due to insufficient cortisol levels, there is loss of negative feedback inhibition in the hypothalamus and pituitary, leading to elevated levels of ACTH and accumulation of steroid intermediates proximal to the enzyme defect.
Consequently, the production of adrenal androgens is increased in girls, resulting in clitoromegaly and sexual ambiguity.
Conversely, androgen production is reduced, and sexual differentiation impaired, in boys with congenital lipoid adrenal hyperplasia (StAR), 3β-HSD2 deficiency, 17OHase deficiency, or p450 oxidoreductase deficiency.
The most common form of CAH, responsible for 95% of cases, is 21-hydroxylase deficiency, resulting from a mutation in the 21-hydroxylase gene. This impairs conversion of 17OHP to 11-deoxycortisol. Therefore, 17OHP is markedly elevated, serving as the clinical marker for diagnosis. 17OHP and its precursors are shunted to androstenedione and DHEA, causing increases in circulating androgens.
Newborn screening: All US states now provide newborn screening for 17OH progesterone. Levels in preterm infants are high (Figure 20-3), making interpretation of screening studies complicated. In the absence of clinical or biochemical findings suggesting CAH, it is reasonable to repeat 17OHP in preterm children every week or two to ensure that levels follow the normal physiologic course. Persistent elevation of 17OHP should prompt a consultation with a pediatric endocrinologist.
Untreated 21OHase deficiency can present with a salt wasting crisis. Deficiencies of cortisol and aldosterone result in hypoglycemia, hyponatremia, hyperkalemia, metabolic acidosis, and hypotension, leading to shock and dehydration. This can also be seen in patients with congenital lipoid adrenal hyperplasia, 3β-HSD2 deficiency, congenital adrenal hypoplasia, bilateral adrenal hemorrhage, pyelonephritis, and obstructive uropathy. Fluid resuscitation with D10 normal saline should be begin immediately, in combination with hydrocortisone IM or IV at a stress dose of 50 to 100 mg/m2 given as a bolus. Thereafter, maintenance IV fluids with dextrose are combined with hydrocortisone 50 mg/m2/d. At stress doses, hydrocortisone has both mineralocorticoid and glucocorticoid effects, thus contributing to the correction of both hyponatremia and hyperkalemia. Chronic treatment involves daily physiologic replacement with hydrocortisone (10 to 15 mg/m2/d), in combination with salt supplements, to promote normal growth, bone maturation, and pubertal development. Fludrocortisone should be given in the setting of hyponatremia or high renin and should be titrated according to electrolytes, blood pressure, and renin levels.
Isolated mineralocorticoid deficiency
Resulting from a mutation in aldosterone synthase, is treated with fludrocortisone and salt supplements; careful monitoring of blood pressure and serum electrolytes is essential.
Defects in mineralocorticoid action (pseudohypoaldosteronism) are managed with salt supplementation and potassium restriction; treatment with hyperkalemia may require the addition of Kayexalate.
Ambiguous genitalia
Table 20-16 provides a broad overview of the possible causes of ambiguous genitalia.
Treatment and long-term management of patients with DSD is complex and should include a multidisciplinary approach: neonatology, pediatric endocrinology, genetics, urology, pediatric surgery, social work, and psychiatry.
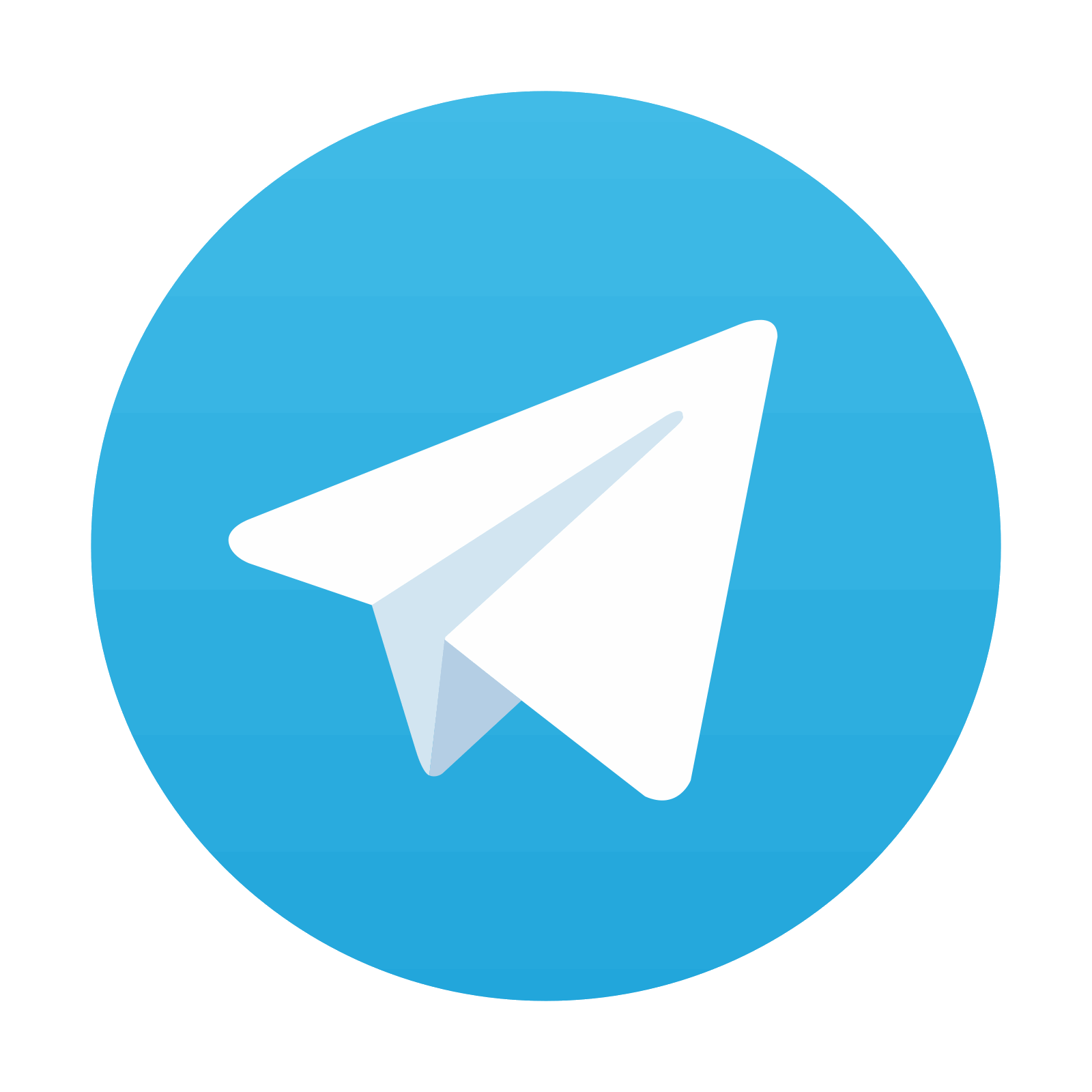
Stay updated, free articles. Join our Telegram channel
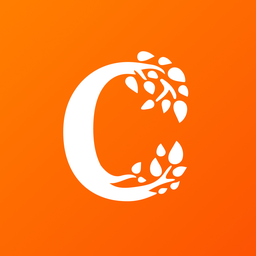
Full access? Get Clinical Tree
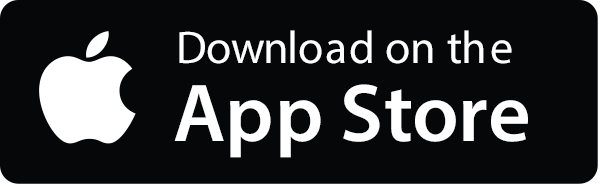
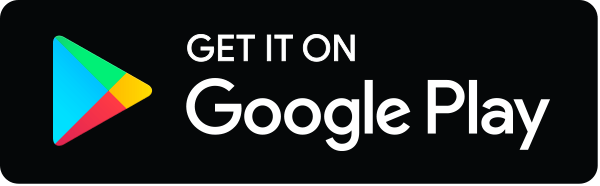