Drug Therapy in the Adolescent
Ian M. Paul
Richard L. Levine
Adolescence is a novel period in human development in which marked physical and physiologic changes occur. These are compounded by the psychosocial development and evolution toward social independence that also occur during these years. These changes affect the way medications and therapeutic strategies are initiated, monitored, and maintained as the individual transitions from childhood to adulthood. Because adolescents share many physiologic similarities with adults, they may not quite be the “therapeutic orphans” (1) that younger children continue to be. Still, they are not simply smaller versions of adults. As recently as the early 20th century, it was suggested in medical literature that 15-year-old children should receive three-fourths of adult doses because they were nearly three-fourths as old as the adult age of 21 years (2). Although science has advanced considerably over the last century, the literature focused on therapeutics for adolescents for many decades revealed a paucity of data relating to the pharmacokinetic and pharmacodynamic changes that occur during this distinctive period of life.
Table 25.1 depicts the various factors that the clinician may consider when designing a therapeutic strategy for an adolescent. These physical, physiologic, and environmental factors influence the often delicate balance between desired and undesired drug effects (3). As the understanding of genetics and the human genome improves, pharmacogenetics will increasingly influence the way diseases are diagnosed and therapeutic strategies are designed for all people, including adolescents (4).
Physical Changes During Adolescence
Physical Growth
Adolescents experience marked changes in their physical size and body composition. Pubertal changes during adolescence coincide with significant increases in height, which alone account for nearly 25% of total adult height (5). Male and female adolescents, respectively, average nearly 10 and 8 increases in height per year during the period of maximal growth rate (6). The normal timing and variability of these increases in height, weight, and pubertal development for both genders have been well established (Figs. 25.1 and 25.2) (7,8,9). The changes in body size often cause difficulty in determining which measure of size should be used for calculating doses of medications. Although most drugs are dosed according to body weight, in other instances the use of body surface area may be more appropriate (10).
Coinciding with the growth acceleration is the significant growth of organs during adolescence (Fig. 25.3), some of which have pharmacologic implications (11,12). For example, urinary clearance of carbamazepine to its metabolites has been shown to be proportional to liver volume relative to body weight (13). Similarly, liver weight was recently shown to be a better parameter for determining warfarin doses than is body weight for growing Japanese children and adolescents (14). In contrast, lorazepam, antipyrine, and indocyanine green did not exhibit age-related changes in clearance based on liver volume when normalized to body surface area (15). Another organ, the small intestine, grows in length and therefore increases its absorptive surface area linearly in relation to body length. Small bowel length has been shown to be the most accurate variable for determining the necessary dose of cyclosporine after liver transplantation for patients younger than 20 years (16,17). Last, the kidneys not only increase in size but also undergo a maturation of their microanatomy that is completed during adolescence (18,19). The growth of the kidney equates with a maximal glomerular volume and absolute number of glomeruli, which both subsequently decline progressively with advancing age (20).
Changes in Body Composition
Many of the important changes in body composition that occur during childhood and adolescence have been described by Friis-Hansen (12,21). During the teenage years, differences in composition between males and females may be more pronounced than during any other period of life. Although males increase the percentage of total body water and reduce the percentage of body fat, females experience the opposite trend (Fig. 25.3). Lean body mass and muscle thickness also increase during adolescence, more rapidly in males than in females, reaching their peak before beginning a progressive decline in early adulthood (22,23). Blood flow to the muscle is also greater during this period and then, too, diminishes gradually with age (24). Similar findings related to tissue perfusion have been reported for the eye (25). The capillary vasculature supplying the musculature also evolves. Adolescents have increased numbers of capillaries per muscle fiber compared with younger children, but less per fiber than in adults (26). Individually and collectively, these changes in body size and composition may influence the pharmacokinetics of drugs and can be part of the source of gender-related differences in drug metabolism, which are seen less in younger children.
Table 25.1 Physical, Physiologic, and Environmental Considerations When Prescribing Drug Regimens for the Adolescent | ||||||||||||||||||||||||||||||
---|---|---|---|---|---|---|---|---|---|---|---|---|---|---|---|---|---|---|---|---|---|---|---|---|---|---|---|---|---|---|
|
Changes in Drug Disposition and Metabolism During Adolescence
During this time of physical growth, numerous physiologic changes occur that affect the way the body processes xenobiotics. This evolution causes differences to varying degrees in each stage of drug metabolism that distinguish this population from those younger and older.
Absorption
Oral Administration
Drugs encounter a complex array of digestive enzymes and hostile environments during passage through the gastrointestinal tract. Although similar to adults and younger children in most regards, the adolescent has certain features that differ from other age groups. Digestion and metabolism for drugs taken enterally begins in the mouth from contact with saliva. The rate of parotid gland saliva secretion markedly decreases in childhood, reaching adult levels during adolescence, a rate that is 10% to 15% of that in a 3-year-old child (27). The gastric pH and rate of gastric emptying, in contrast, are relatively constant outside of the neonatal period (28). Distal to the stomach, the small intestinal transit time is also known to be relatively constant, suggesting that the velocity of travel increases as children grow (29,30). In contrast, colonic motility during adolescence is reduced compared with that in younger children (31). Finally, the activity of some enzymes involved in extrahepatic biotransformation located in the intestinal mucosa may differ during this time of life, but the activity of enzymes released by the pancreas does not (32,33).
These physiologic changes may account for some of the variability in bioavailability of drugs after oral administration and for the differing rates of absorption seen during adolescence (34). Just as calcium absorption increases during puberty to account for metabolic needs, the amount of a given drug that is absorbed by the intestine may vary with age (35). For example, lower serum levels of penicillin were found in older children and adolescents compared with infants after oral administration (36). This was partially attributed to a reduced absorption in that population. Next, inconsistent absorption of sustained-released theophylline within and between subjects including adolescents has resulted in variable serum levels (37). In addition to the amount absorbed, significantly slower absorption of midazolam has been demonstrated for children between ages 12 and 16 years when compared with those younger than age 12 (38).
Another factor that may account for differences is the change in gut flora that occurs with age. This evolution can influence the absorption of medications as demonstrated by the increasing ability of these bacteria to metabolize digoxin throughout childhood (39). The adult pattern of metabolism is not reached until adulthood, reflecting the development of enzymes such as β-glucosidase and reductases secreted by these intestinal organisms (40).
Topical Application
As for absorption through the skin, the major change between adolescents and other age groups is the surface area for potential exposure, which proportionally decreases with increasing body size. The physiology and percutaneous absorption of drugs, however, appear to be relatively constant throughout life (41). One potential source of variability may be the known reduction in cutaneous blood perfusion between childhood and adulthood, though limited research has been performed to demonstrate the effect of this variable (42).
Distribution
Body Composition
Changes in drug distribution throughout the body that occur during adolescence can largely be attributed to the physical growth and redistribution of body compartments. For example, lipophilic drugs, such as many psychoactive medications, may have shorter half-lives in adolescents than in younger children and adults because of the reduction in body fat that generally occurs during these years (43). Similar results have been seen for lipid-soluble anesthetic agents such as thiopental and propofol, the latter of which was shown to require increased doses for induction of anesthesia for adolescents and those with greater lean body mass than for older, fatter patients (44,45). In contrast, theophylline was shown to have a longer half-life in adolescents related to an increase in lean body mass (46).
Protein Binding
Changes in plasma proteins also influence drug distribution and are known to vary with age. Beginning in adolescence, there is a progressive decline with age of serum levels of albumin but an increase of α1-acid glycoprotein (47). This causes differences in affinity for medications after adolescence, resulting in increased amounts of some unbound drugs such as desipramine, salicylic acid, phenytoin, and lidocaine but reduced amounts of other unbound drugs, including chlorpromazine (47,48). In contrast, seizure medications such as valproic acid and carbamazepine that have a narrow therapeutic window fortunately do not appear to have changes in protein binding among younger children, adolescents, and adults (49,50,51,52).
Metabolism and Clearance
During the pubescent years, the liver, where the majority of drugs are metabolized, undergoes significant maturational changes. In addition to an increase in size, there are also changes in the enzymes and metabolic pathways located within the liver that are responsible for the processing of medications. During this time, it is likely that hormones involved with growth and sexual development have an influence on drug metabolism (53). Though mechanisms for hormonal influences on drug metabolizing enzymes are incompletely understood, it is notable that in general, the developmental pattern of enzymatic activity is inversely related to levels of hormones involved in the hypothalamic-pituitary-gonadal axis and corresponding pubertal stage (9). This inverse relationship is most obvious during the two life stages with the greatest levels of hormonal change, early infancy and adolescence.
Phase-1 Reactions
The cytochrome P450 (CYP) enzymes are responsible for the metabolism of many xenobiotics via oxidation and reduction reactions primarily within the liver. Although these enzyme systems have been shown to mature at varying times throughout development, there are contrasting opinions as to whether these changing activities account for the differences in drug clearance seen among different age groups (54,55,56). Most agree though that age should be considered an important variable for any evaluation of the CYP system (57). A database recently designed to examine the age-related pharmacokinetic variability of 45 drugs found that the CYP enzymes, individually and collectively, did not contribute to overall variability between adolescents and adults, although significant differences did exist between teenagers and younger age groups (Fig. 25.4) (58). Alternatively, one investigation demonstrated reduced clearance of antipyrine after adolescence (59). Because antipyrine is a widely used marker for overall hepatic drug-metabolizing capacity due to the numerous CYP enzymes involved in its metabolism, this is particularly noteworthy (60). Confounding the issue further, both antipyrine and another drug used as a marker for similar reasons, indocyanine green, demonstrated weight-adjusted clearance values that correlated significantly with age from childhood through adolescence into adulthood (61,62). These correlations disappeared, however, when these values were adjusted for body surface area.
The need for continued investigation in this area is highlighted by several studies that indicate that levels of individual enzyme activity do vary with age including during adolescence. For example, a frequently cited study employed isotopically labeled CO2 to evaluate cytochrome P450 metabolism of caffeine (63). The (3–13C-methyl) caffeine breath test described in this investigation demonstrated that clearance mediated by CYP1A2 declined to adult levels during adolescence, but that girls achieved this earlier in puberty (Tanner stage 2) than did boys (Tanner stage 5). Similarly, theophylline clearance was found to diminish linearly with increasing age for participants aged 1 to 30 years (64). Proton-pump inhibitors such as omeprazole and lansoprazole, metabolized primarily by CYP2C19, demonstrated a reduction in clearance during adolescence and adulthood when compared with younger children (65,66). Similar results were shown for the metabolism of phenytoin by CYP2C9 and chlorpromazine by CYP2D6 (67,68). Furthermore, two investigations reported that carbamazepine is converted to its epoxide metabolite by CYP3A4 at a faster rate in younger children than in older children and adolescents, with a resultant higher ratio of metabolite to parent compound existing in the younger age groups (69,70). Finally, a progressive decline in cyclosporine clearance by CYP3A4 from childhood through adolescence into adulthood has also been demonstrated, which remained even after adjustment for body surface area (71).
Although hepatocytes are the primary location where these enzymes metabolize drugs, they are also abundant in other tissues (72). Within enterocytes, CYP3A4 levels were recently shown to increase with age throughout childhood into adolescence (73). This potentially could modify drug bioavailability after oral administration.
Phase-2 Reactions
The ontogeny of nonoxidative metabolic reactions, which include acetylation, sulfation, glucuronidation, and glutathione conjugation, has received considerably less attention in adolescent pharmacology despite their significant contribution to the metabolism and clearance of xenobiotics. In contrast to the results described for the CYP enzymes, the pharmacokinetic database described previously was indeed able to detect overall age-related changes in phase-2 reactions, notably glucuronidation, between adolescents and adults (Fig. 25.5) (58). Lorazepam, morphine, valproic acid, and zidovudine were some of the substrates included in this pharmacokinetic analysis.
From individual analyses, when normalized for body surface area, lorazepam was shown to have increased clearance with age through adolescence into adulthood (61). The opposite result was demonstrated for busulfan, where enterocyte glutathione S-transferase activity declined with age through adolescence (74). Conflicting with the collective and individual findings, zidovudine when examined alone was not shown to have age-related variability in conjugation and overall metabolism among young children, adolescents, and adults (61,75).
For acetylation, the presence of a bimodal phenotypic distribution of metabolism has emerged, with individuals classified as either fast or slow acetylators (76). This distribution appears to vary as a function of age, with an increased prevalence of fast acetylators younger than 15 years compared with older individuals (77). During childhood, the distribution also changes, with the frequency of fast acetylators increasing until age 4 and then remaining relatively constant throughout the remainder of childhood and adolescence (78).
Importantly, there are also age-related changes in the predominance of specific metabolic pathways. Overall, the rate of acetaminophen clearance remains constant with age. In contrast to adults and adolescents, however, for young children, sulfation, not glucuronidation, is the principal method of conjugation for both acetaminophen and salicylamide (79,80). It is not until approximately age 12 that the adult pattern develops where glucuronidation predominates (79).
Elimination
The kidney is responsible for the elimination of the majority of water-soluble compounds and fat-soluble substrates that are metabolized to hydrophilic agents, though other organs such as the lung, biliary tract, intestines, and skin contribute to the excretion of drugs (81). Kidney function as measured by the glomerular filtration rate is relatively constant outside of infancy. Another important function of the kidney, tubular reabsorption, also varies little throughout childhood into adulthood and is not influenced by pubertal stage (82).
One renal parameter that does appear to develop throughout childhood and adolescence is tubular secretion, which was shown to decline with advancing age for uric acid (83). When this function was tested using digoxin as the substrate, similar results were obtained (84,85). The net tubular secretion of digoxin declined during adolescence to amounts seen in adults, but appeared to better correlate with Tanner staging and therefore sexual maturity than with age alone. Methotrexate, which is excreted by the kidney via glomerular filtration and tubular secretion, also shows reduced clearance as a function of age through adolescence (86). Tubular secretion may also account for the differences in renal ceftriaxone and theophylline clearance that occur during adolescence (87,88).
Therapeutic Considerations
Differences between Sexes
Because the physical and hormonal disparities between males and females are much greater during adolescence than in other parts of childhood, it is at this age that pharmacokinetic variability between the sexes may first become prominent. Differences may affect nearly all aspects of drug disposition and metabolism. Beginning with absorption, intestinal transit time differs between sexes, which may influence the bioavailability of some drugs when administered orally (89). As for distribution, the significant differences in lipid and lean body compartments between sexes (Fig. 25.6) can affect the pharmacokinetics of many drugs, particularly those that are lipophilic. Numerous sex-related differences in drug metabolism have also been described with distinctive activity levels for some, but not all phase-1 and -2 enzymes (90,91). Because the genes for these enzymes are located on autosomal chromosomes, it is presumed that differences in activity likely result from interactions with endogenous hormones (92). Finally, the influence of the menstrual cycle on pharmacokinetics and pharmacodynamics is controversial, with conflicting data supporting an effect and a lack thereof (93). Although the majority of sex- or menstrual-related differences are minor, there are instances when the disparity can cause significant effects. A frequently cited example is the greater prolongation of the QT interval for women given cardiovascular medications than for men (94,95). The associated increased risk for torsades de pointes may also be influenced by the menstrual cycle (96).
Contraception
The extraordinary changes that occur during adolescence include the development of a sexual identity. In addition, adolescents have feelings of invulnerability as part of their normal development. This combination can lead to significant risk-taking behavior by adolescents including sexual activity.
The early initiation of teenagers into sexual activity exposes them to a host of health problems such as unintended pregnancy and sexually transmitted diseases (STDs). The United States has the highest teen pregnancy rate of all developed countries. This rate had been declining from 1991 through 2005 (97,98). At least part of this decline was the result of available information and access to appropriate contraception. The birth rate for the youngest teens, aged 10 to 14 years, continued this decline in 2006. Unfortunately, the birth rate for teens 15 to 19 years increased 3% in 2006 (99).
There are many effective forms of contraception for adolescents, though none are completely effective at preventing pregnancy or STDs. These include nonhormonal/barrier methods and hormonal methods. A general rule for contraception for adolescents is that the use of two methods simultaneously is better than one alone, and one method should always be a condom to protect against the spread of STDs including the human immunodeficiency virus (HIV).
Contraception efficacy is measured by its success or failure rate (100). This includes its theoretical effectiveness with correct and consistent use and its actual or typical use effectiveness rate (98,100). Because of various developmental and social factors, teens generally have lower actual use efficacy rates (100).
The nonhormonal/barrier methods include the condom, the female condom, the diaphragm, and the cervical cap. Timing ovulation via the rhythm method to avoid pregnancy, withdrawal before male ejaculation, and vaginal douching after intercourse are not effective forms of contraception.
The last several decades have seen an evolution of hormonal methods of contraception, with numerous currently available options. These include oral, injectable, and implantable contraceptives; the intrauterine device (IUD); emergency contraception; and newer forms of contraception including the contraceptive patch and the vaginal ring.
Oral Contraceptives
Oral contraceptives include the combined oral contraceptive (COC) pill and the progestin-only contraceptive pill (Table 25.2). There are two forms of COCs, including fixed dose and multiphasic pills. All of the COCs contain an estrogenic component and a progestational component. Most COCs contain 21 days of combined estrogens and progestin with 7 days of inert pills during which withdrawal bleeding occurs. One formulation (Mircette™) contains only 2 days of placebo pills to limit estrogen withdrawal symptoms, whereas others (Loestrin® Fe, Estrostep® Fe) contain iron during the week without hormones (98,101). The current estrogen component of most COCs is ethinyl estradiol at a dose of 20 to 35 μg per tablet (98,102,103). Mestranol is found in a small number of pills and is metabolized to ethinyl estradiol (98,102,103). The progesterone component includes the older progestins such as norethindrone, norethindrone acetate, norgestrel, levonorgesterol, and ethynodiol diacetate, but the potencies of these agents are somewhat controversial (98,102,103). In general, norethindrone, norethindrone acetate, and ethynodiol diacetate are considered equally potent; norgestrel is 5 to 10 times more potent, and levonorgesterol is 10 to 20 times more potent (103). The newer progestin pills contain norgestimate and desogestrel, and there is some evidence that the new progestins have a greater amount of the desired progestational effect and fewer undesirable androgenic side effects (98,102,103). Two additional COC that are relatively new (Yasmin® and Yaz®) contain drospirenone, which is an antimineralcorticoid derivative of spironolactone developed to limit COC-associated weight gain (103,104). It also has some antiandrogenic effects and might be a good choice for patients with hyperandrogenism such as polycystic ovary syndrome (105). In addition, there are two new extended cycle oral contraceptive on the market called Seasonale® and Seasonique™, which contains ethinyl estradiol and levonorgesterol in a 91-day cycle—84 active pills with 7 placebo pills or very low estrogen pills. These are helpful in patients with menstrual-related medical issues such as menstrual migraines (105). There are no studies in adolescents on the newest extended cycle pill, Lybrel®, which is given continuously, 365 days per year.
Mechanism of Action
The mechanism of action of all of the COCs includes (a) inhibition of ovulation; (b) modification of cervical mucous to make it more viscid, thus limiting spermatic motility; (c) alteration of the endometrium to make it unsuitable for implantation; and (d) reduction of fallopian tubule motility (102,103). The theoretical effectiveness of COCs is 99.9%, but the actual efficacy ranges between 92% and 95% (92% for adolescents) (98,100,102,103). A number of measures are utilized to increase adolescent compliance including comprehensive education about the pills and their health benefits, counseling regarding the logistics of using the pills, and close follow-up (100,102,103).
Health Benefits
It is very important to consider the health benefits of COCs for adolescents. First, there are the menstrual benefits. COCs have an antiproliferative effect on the endometrium and thus reduce menstrual flow, the duration of menstruation, and subsequently the occurrence of menstruation-related anemia (98,102,106). In addition, menstrual periods are more regular, and midcycle pain, or Mittelschmerz, is reduced because ovulation is inhibited (102,106). Young women with anovulation and dysfunctional uterine bleeding have significantly more regular, predictable cycles as well (102,106,107). Dysmenorrhea is also markedly reduced in young women with use of COCs (102,106,108). In fact, many young women primarily use COCs for this purpose. COCs produce this effect through a reduction in the production of prostaglandin F2α by decreasing the proliferation of the endometrium (106,108). Polycystic ovary syndrome is often treated with COCs using continuous dosing for 2, 3, or more months without the placebo weeks (106). This serves to limit androgen excess by decreasing ovarian androgen production via inhibition of luteinizing hormone secretion (106). In addition, COCs increase sex hormone-binding globulin, which decrease free testosterone levels (106).
Table 25.2 Oral Contraceptive Formulations Currently Available in the United States | ||||||||||||||||||||||||||||||||||||||||||||||||||||||||||||||||||||||||||||||||||||||||||||||||||||||||||||||||||||||||||||||||||||||||||||||||||||||||||||||||||||||||||||||||||||||||||||||||||||||||||||||||||||||||||||||||||||||
---|---|---|---|---|---|---|---|---|---|---|---|---|---|---|---|---|---|---|---|---|---|---|---|---|---|---|---|---|---|---|---|---|---|---|---|---|---|---|---|---|---|---|---|---|---|---|---|---|---|---|---|---|---|---|---|---|---|---|---|---|---|---|---|---|---|---|---|---|---|---|---|---|---|---|---|---|---|---|---|---|---|---|---|---|---|---|---|---|---|---|---|---|---|---|---|---|---|---|---|---|---|---|---|---|---|---|---|---|---|---|---|---|---|---|---|---|---|---|---|---|---|---|---|---|---|---|---|---|---|---|---|---|---|---|---|---|---|---|---|---|---|---|---|---|---|---|---|---|---|---|---|---|---|---|---|---|---|---|---|---|---|---|---|---|---|---|---|---|---|---|---|---|---|---|---|---|---|---|---|---|---|---|---|---|---|---|---|---|---|---|---|---|---|---|---|---|---|---|---|---|---|---|---|---|---|---|---|---|---|---|---|---|---|---|---|---|---|---|---|---|---|---|---|---|---|---|---|---|---|---|
|
Other health benefits include (a) a reduction in the risk of benign breast disease including fibroadenomas and fibrocystic disease (109); (b) treatment of mild-to-moderate cystic acne (110,111); and (c) protection against dysfunctional uterine bleeding, ectopic pregnancy, pelvic infections, ovarian cancer, and endometrial cancer (102,106,107,111,112,113,114).
Effects on Metabolism and Potential Adverse Effects
Potential adverse effects of COCs include medically significant complications generally related to oncogenic potential, circulatory complications including the risk of thromboembolism, hepatic changes, and lipid abnormalities.
The potential impact of COCs on the development of breast cancer is not clear and has become controversial. Studies have produced conflicting results regarding the incidence of breast cancer in patients who have taken COCs (102,103,115,116). For example, two recent studies indicated a slight increase in the risk of breast cancer in long-term users of COCs (117,118). One of these studies found that the new, lower estrogen pills imparted a lower risk than earlier, higher potency COCs (118). However, another recent investigation found no association between current or former COC use and the risk of breast cancer (119).
There is more certainty regarding the lack of association between COCs and other forms of cancer. As previously mentioned, studies indicate that COCs have a protective effect on the development of endometrial cancer by preventing unopposed stimulation by estrogen (102,103,115,116). In addition, patients who have taken COCs have a decreased incidence of ovarian cancer, and there seems to be no effect on the development of cervical cancer (102,103,111,112,113,115,116).
There are also a number of consistent metabolic effects of COCs. One of the most significant is the effect on the extrinsic clotting pathway. Fibrinogen and factors I, V, VII, VIII, and X are increased, and fibrinolytic and anticoagulation factors are increased as well (102,103,115). The balance of these changes can increase the risk of thromboembolism with COC (102,103,115). There is a clear relationship between the dose of estrogen in the COC and the risk of thromboembolism, with higher doses producing an increased risk (101,102,103,115,116). The overall incidence of venous thromboembolism is 15 to 30 per 10,000 woman-years for the low-dose pill formulations, but is known to be greater for patients with the factor V Leiden gene mutation (101,102,103,115,117). As for the risk of venous thrombosis with the new progestins desogestrel and gestodene, some epidemiologic studies suggested that they are associated with a higher risk, whereas other researchers claimed that these studies are flawed and that there is no actual increased risk (101,103).
Fortunately, there does not appear to be an increased risk of cerebrovascular accidents or myocardial infarctions in patients on low-dose COCs (102,103,115,116). Smoking does, however, increase the risk of all types of cardiovascular disease with use of COCs (98,102,103,115,116). Clearly, practitioners should discourage smoking for all adolescents, but nevertheless it is not considered a contraindication to the prescription of COCs for teens (98,102,103).
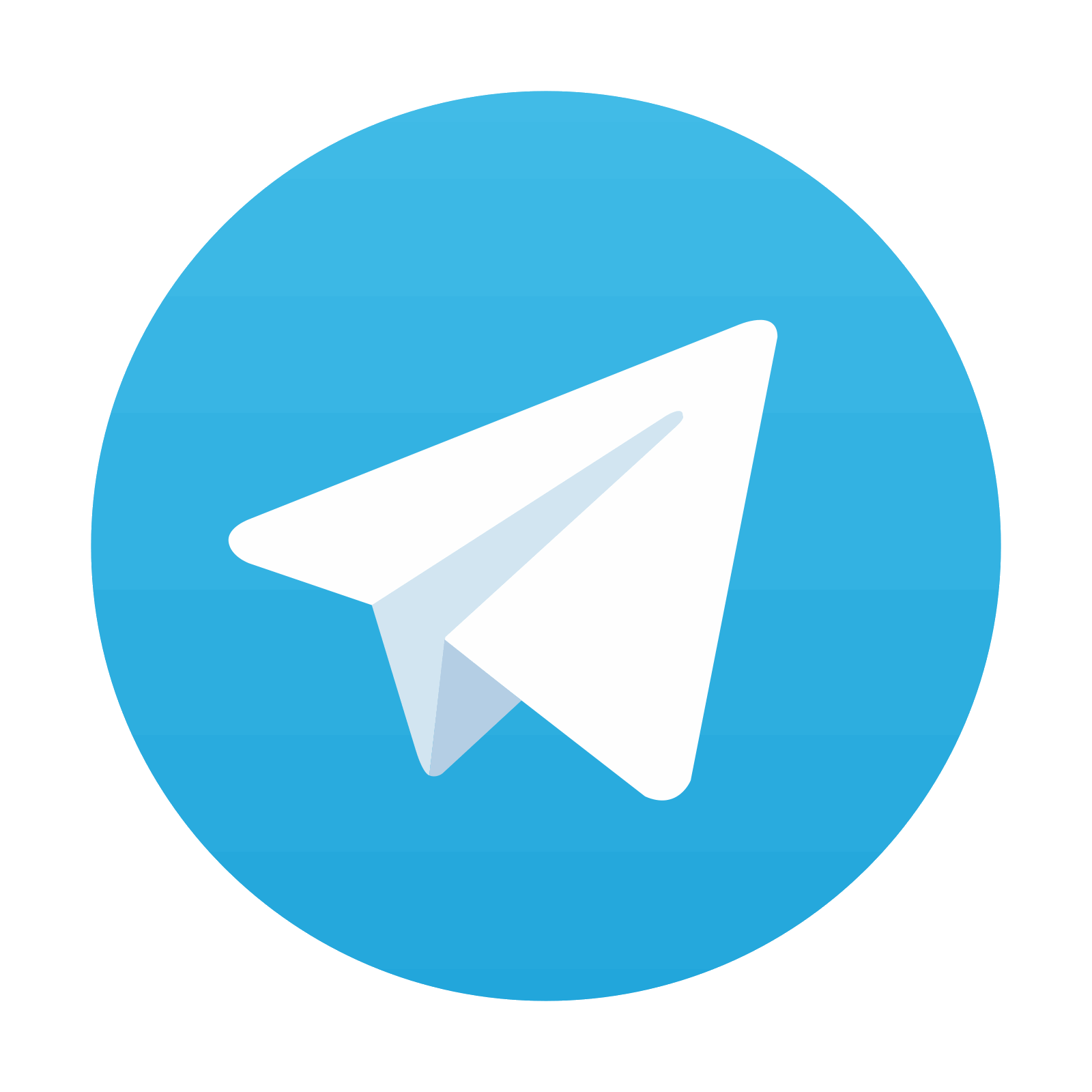
Stay updated, free articles. Join our Telegram channel
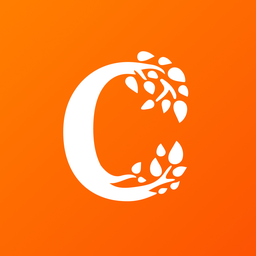
Full access? Get Clinical Tree
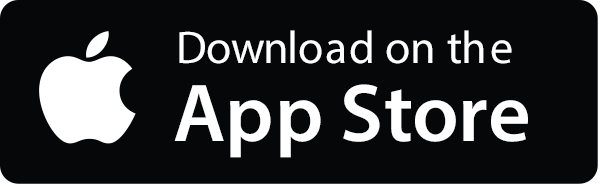
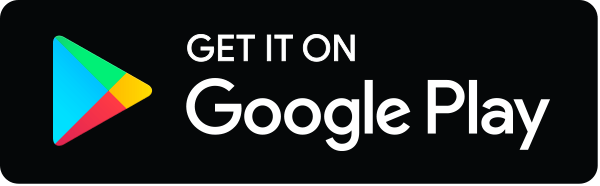