(7)
Department of Respiratory Care, Seattle Children’s Hospital Foundation, Research Institute, 4800 Sand Point Way NE, Seattle, WA 98105, USA
Educational Goals
-
Understand the basics of aerosol characteristics.
-
Describe the factors that affect aerosol delivery during mechanical ventilation.
-
Describe the basic function of pneumatic nebulizers, vibrating mesh nebulizers, and metered-dose inhalers.
-
Understand the relative effectiveness of these devices at delivering aerosols to the lung during mechanical ventilation.
-
Describe the benefits of and clinical response to aerosolized bronchodilators during mechanical ventilation.
30.1.1 Introduction
Humans have been using the inhalational route for administering medication, both therapeutic and recreational for many centuries (Anderson 2005). The term inhaler was first used in the English literature in the eighteenth century (Sanders 2007; Rubin 2010). A great deal of creativity has gone into the naming of inhaler devices, some of which have deservedly been cast onto the ash heap of history including the volatilizer, varoma, vapo-haler, spirit burner, vapo-cresoline, pillow inhaler, atomizer, inspirator, pipe medicator, pulverisateur, and the ammoniaphone. 1 At one time, various forms of inhaled tobacco smoke were thought to be therapeutic. In 1571, a Spanish doctor named Nicolas Monardes wrote a history of medicinal plants of the new world, in which he claimed that tobacco could cure 36 health problems.2 Cigarettes were developed for the treatment of asthma that were made of an anticholinergic substance and were available for purchase up until the 1970s (Elliott and Reid 1980). This chapter will focus on the administration of medications via inhalation during mechanical ventilation of infants and children.
The inhaled route for administering medications has significant advantages over other routes. These include (Le Brun et al. 2000):
-
Reduced first pass effect: This term refers to reduction in the concentration of a drug before it reaches the systemic circulation. Once swallowed, a drug is absorbed by the digestive system. It passes through the liver before it reaches the systemic circulation. Often, most of the drug is metabolized in the liver, some to the point where only a small percentage of the ingested drug remains, reducing bioavailability.
-
Shorter lag time: Since most aerosolized drugs are administered directly to the site of action when inhaled, there is generally reduced lag time between delivery and the start of the action of the drug. With bronchodilators this results in a nearly immediate effect.
-
Fewer systemic side effects: Again, since the aerosolized drugs are typically administered directly to the site of action, there are typically fewer systemic side effects.
Some of the technical aspects, advantages, and limitations of creating and inhaling therapeutic aerosols in ventilated children are related to the anatomy of the pediatric airway and lung and the size of the equipment being used. By the 17th week of gestation, the full number of generations of conducting airways has been established (Wohl 2006). The implications of this are very important for the efficacy of inhaled medication in children. These airways are significantly narrower in diameter than in adults. The passage through and deposition of aerosols in these airways are substantially different than in adults. This is due, in part, to the fact that most aerosol-generating technology has been developed for use in adults. In addition, much of the performance data and conventional wisdom about the ability of various devices to deliver aerosols to the middle and lower respiratory tract are predicated on the behavior of aerosols in the adult lung. Table 30.1 compares some basic structural and functional differences between the lungs of adults and infants.
Table 30.1
Comparison of infant and adult lung and airway characteristics
Infant/adult |
Infant |
Adult |
---|---|---|
Trachea, diameter |
4 cm |
20 cm |
Trachea, length |
5–6 cm |
10–12 cm |
Tidal volume |
20–40 mL |
400–700 mL |
Respiratory rate |
30–40/min |
12–14/min |
Inspiratory flow rate |
≤100 mL/s |
500 mL/s |
Bronchodilators are the most commonly administered drug via the inhaled route in ventilated patients. For the purposes of analyzing the best way to give an aerosol, the following discussion will focus on the administration of inhaled bronchodilators. Many other drugs are available or under development in formulations that can be administered via the inhaled route, including antibacterials, antivirals, antifungals, immunosuppressive drugs, nonsteroidal anti-inflammatory drugs, surfactants, prostaglandins, mucolytics, antitussives, gene therapy vectors, insulin, heparin, ergotamine, calcitonin, human growth hormone, sildenafil, vaccines, morphine, and fentanyl (Rubin 2010). Often, the type of drug being aerosolized dictates the use of different kinds of aerosol-generating devices (Waldrep and Dhand 2008). Liposome encapsulation is emerging as a potentially better way to formulate drugs for aerosol deposition in the lungs. Liposomes are tiny vesicles or sacs that can be formed around solution to be inhaled. The liposomes create a more sustained response of the drug and fewer side effects (Dhand 2004). Newer formulations of drugs that are liposome encapsulated will require specific and sometimes different aerosol-generating technology (Waldrep et al. 1994; Saari et al. 1999; Zaru et al. 2007; Gagnadoux et al. 2008; Elhissi et al. 2007).
30.1.2 Characteristic and Behavior of Aerosols
An aerosol is a group of solid or liquid particles suspended in a gaseous medium. The aerosols generated by clinical devices are composed of many different sized particles and referred to as heterodisperse aerosols (or polydisperse). The distribution of particle sizes is an important characteristic that affects the ability of the aerosol to achieve therapeutic goals. One term used to describe distribution of the size of aerosol particles is mass median aerodynamic diameter (MMAD) which can be defined as the particle diameter that divides the distribution of particle sizes in half by mass. In other words, half of the mass of an aerosol is made of particles larger than the MMAD and half the mass is made up of smaller particles.
For a therapeutic aerosol like bronchodilator therapy to be effective, the particles must transit the aerosol-generating device, connecting tubing/device, and the upper airway, finally coming out of suspension and depositing on the walls of the medium and small airways. Therapeutic aerosols range in diameter from 0.1 to >10 μm. Particles >5 μm tend to deposit in the medium and larger airways, while particles <1.0 μm are so small as to be exhaled without depositing in the airway. For adults, aerosol generators are best that can produce particle size distributions with a high proportion of the particles in this respirable range (1–5 μm) (Heyder et al. 1986; Balásházy et al. 2007).
But these axioms about aerosol deposition by particle size in various parts of the airway are all based on the anatomy of the adult airway. It is not unreasonable to assume that the most efficacious distribution of particle sizes for infants and children is smaller than that postulated for adults, but I have never seen any literature that has analyzed or studied this issue.
When a suspended aerosol particle moves near enough to the walls of the airway, it comes out of suspension and deposits. It is thought that this occurs at a distance from the wall of the airway of ≤ than one radius of the particle (Kleinstreuera et al. 2008). Mechanisms of this deposition include inertial impaction and gravitational sedimentation. Inertial impaction results when particles are moving in one direction in the gas stream through airways that are tortuous. The physical laws of motion govern the movement of these particles such that their inertia (or linear momentum) tends to carry them in one direction. The linear momentum of particles is the product of their mass times their velocity. Particles tend to continue in a straight line until acted on by another force or impacting the wall of the airway, where they deposit out of suspension. This effect will be more pronounced with larger particles because of their higher linear momentum, which explains why larger particles tend to deposit sooner, in the upper airways. Higher inspiratory gas flow rates should increase inertial impaction, again because particles moving at a higher rate have an increased linear momentum.
Gravitational sedimentation occurs when particles reside long enough in the airway to settle out and touch the walls of the airway when acted on by gravity. This is why breath holding can increase aerosol deposition in the airways, because during this pause in flow, the linear momentum of particles is lower and the effects of gravity can draw the particles into the walls of the airway. Lower flow rates can also enhance gravitational sedimentation, again because the suspended aerosol particles will have a lower linear momentum. This lower velocity of gas allows more transit time of the particles in the airway and more gravitational sedimentation is possible.
Aerosol characteristics are also affected by the phenomena of coalescence. When two aerosol particles come into relatively close proximity with one another, they combine to form a larger particle. Coalescence tends to happen when particles come within a distance of one another less than 25 diameters of the particle sizes. Coalescence is increased during higher (more turbulent) flow rates because more turbulent flow will increase the number of particle collisions.
The combined effects of inertial impaction and coalescence (which creates larger particles from smaller ones) can cause increased relative amounts of an inhaled aerosol delivered to ventilated patients to deposit (1) on the walls of the tubing and chambers connecting the aerosol to the artificial airway, (2) in the artificial airway, and (3) in the larger and medium airways. Deposition profiles like this are generally not very therapeutic. As previously stated these phenomena are enhanced when inspiratory flow rates and respiratory rates are higher. They are also enhanced when both the artificial and natural airways are smaller. This makes delivery of inhaled aerosols to ventilated infants and small children very challenging, and many types of delivery systems result in very little of the total aerosolized dose actually getting through the equipment and the artificial airways to the parts of the patient lungs where the drug can be effective (Fink 2004). It is reported that pulmonary deposition of nebulized aerosols in neonates may be as low as 1 % of the nominal dose, compared to 8–22 % in adults (Rubin and Fink 2001).
There is emerging information that the nature of pulmonary disease affects the deposition of aerosols into different parts of the lung (Apiou-Sbirlea et al. 2010). This is because various degrees of airway constrictions, blockages, or remodeling cause disruption and redirection of flow into various parts of the lung. In part, this information has been developed using sophisticated mathematical modeling, and the overall complexity of the modeling makes straightforward application of this information at the bedside to improve aerosol delivery difficult. But in general, aerosol delivery will be improved by the application of pulmonary toilet procedures before aerosolized medications are administered to patients in order to remove any blockages or narrowing of the airways related to retained secretions.
Many in vitro studies have been published, testing the delivery of aerosols during mechanical ventilation of infants and children (Rubin and Fink 2001; Cameron et al. 1991; Benson et al. 1991; Everard et al. 1992; Arnon et al. 1992; Grigg et al. 1992; Coleman et al. 1996; Garner et al. 1994, 2000, 2002; Lugo et al. 2001; Pelkonen et al. 1997; Wildhaber et al. 1998; Avent et al. 1999; Habib et al. 1999; Dubus et al. 2005). Generally, these studies use neonatal and pediatric lung models that compare the output of delivery devices like nebulizers and metered-dose inhalers (MDI) to the amount of the aerosol that can be recovered distal to the endotracheal tube. Fink published an excellent analysis of these studies (Fink 2004). For various types and brands of nebulizers, airway deposition of the emitted aerosol dose has been reported to range from 0.02 to 8.0 % with a mean of 2.01 ± 2.01 % (±standard deviation). For MDIs deposition was reported from 0.12 to 15.5 %, with a mean of 4.70 ± 4.70 %. Figure 30.1 is derived from analysis of these studies and shows the distribution of drug delivery in these various lung models with nebulizers versus MDIs.
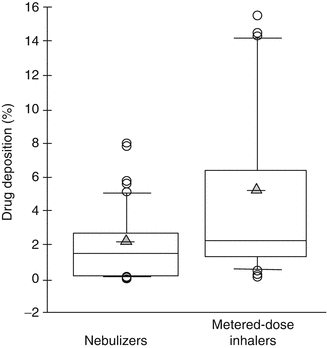
Fig. 30.1
Distribution of summarized results from 13 published in vitro studies of drug deposition in various neonatal and pediatric lung models during mechanical ventilation comparing the performance of nebulizers and metered-dose inhalers. Box plots represent the percent drug deposition reported in each study. Some studies reported several deposition rates because they tested various devices and/or delivery configurations. Circles represent aerosol particles of various sizes. Shaded triangles represent the arithmetic mean (Adapted from the work of Fink (2004))
Consider the very large range and variation of device performance for both nebulizers and MDIs. Coefficients of variation for both distributions are 100 %. Variation notwithstanding, MDIs appear to be better devices than pneumatic nebulizers for delivering aerosolized medication during mechanical ventilation for reasons that will be described below.
Why such large variation and poor overall drug delivery in these studies? Because there are many factors that affect the efficacy of inhaled drug delivery systems during mechanical ventilation in pediatric patients. These include (1) the type of aerosol generator used, (2) the location or placement of the aerosol generator in the ventilator circuit, (3) the use of humidified gas, and (4) the patients’ ventilatory parameters.
30.1.3 Factors Affecting Aerosol Delivery During Mechanical Ventilation
30.1.3.1 Types of Aerosol Generators Used
There many different types and brands of aerosol-generating devices. The overwhelming majority of all inhaled medications during mechanical ventilation are delivered using either nebulizers or MDIs, but at one time, the majority of medication delivery during mechanical ventilation of infants in the USA was done with pneumatic nebulizers, (Salyer and Chatburn 1990) but practice is evolving and MDI’s use is increasing (Ballard et al. 2002).
Nebulizers can be broadly categorized into two types: (1) pneumatic nebulizers and (2) vibrating mesh nebulizers.3 For use during mechanical ventilation, MDIs are generally used in one of three ways: (1) with an in-line adapter, (2) with a spacer, and (3) with a valved holding chamber.
30.1.3.2 Pneumatic Nebulizers
Pneumatic (or jet) nebulizers employ the Venturi effect to create an aerosol. When a gas stream is directed from a wider to a narrower orifice tube, the velocity of the gas increases, and its pressure drops. If the size of the narrowing is properly calibrated, a capillary tube connected to a fluid reservoir can be inserted in the narrowed orifice and fluid will be drawn into the gas stream by this reduced pressure and broken into suspended particles (an aerosol). Figure 30.2 is a conceptual schematic representation of the design of pneumatic nebulizers. The Venturi effect typically creates a heterodisperse aerosol that has very high variation in particle sizes and limited proportion of particles in a range suitable for deposition in the lungs. This is remedied by the use of baffles in the nebulizer which are designed so that larger particles will impact on the baffles and recovered in the nebulizer for re-nebulization, leaving the remaining aerosol composed of a higher proportion of aerosol particles in the respirable range.4

Fig. 30.2
Conceptual schematic representation of a typical pneumatic nebulizer. Circles represent aerosol particles of various sizes. Note the position of the baffles. The distribution of particle sizes is greatly improved as the aerosol passes through the baffles where many larger particles impact the baffles and deposit. The design of the nebulizers is such that the aerosol that deposits on the baffles drains back into the nebulizer fluid chamber and is re-nebulized
Not all pneumatic nebulizers are created equal, although this reality is not yet fully appreciated by many clinicians. Considerable performance variation has been reported between brands of the same types of nebulizers in the past (Alvine et al. 1992; Hess et al. 1996) and more recently (Berg and Picard 2009). This variation takes the form of differences in the aerosol characteristics, such as the respirable range of particles created by these nebulizers. Additionally, there is almost certainly variation in performance within the same brands/models of nebulizer owing to imprecision in the manufacturing process, although I am unaware of any significant work published that quantifies this variation. Figure 30.3 describes variation between brands of pneumatic nebulizers in the distribution of particle sizes.
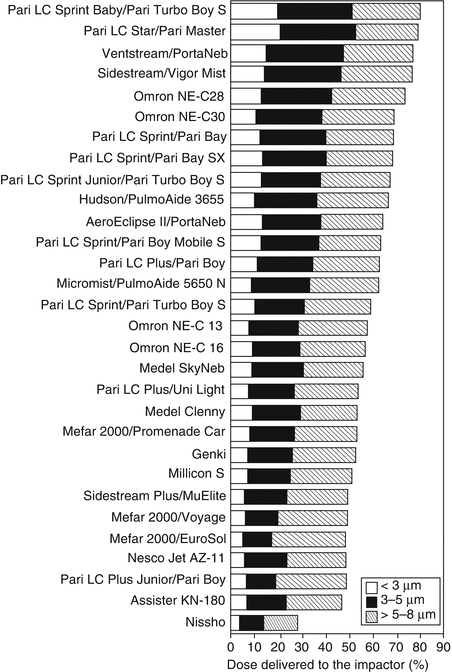
Fig. 30.3
Droplet size distribution of budesonide inhalation suspension up to 8 μm in various brands of pneumatic nebulizers. Three units of each brand were tested. The results are expressed as a percentage of budesonide mass delivered to the impactor (Used with permission from Berg and Picard (2009))
Variable nebulizer performance and application may help to explain some of the lack of response to bronchodilators so often seen during treatment of ventilated patients. In many cases, it may be that the patient is getting very little of the aerosolized medication delivered to the airways. It can also explain the wide variation and generally poor findings reported in the literature for various studies of delivery and/or deposition of aerosolized medications.
30.1.3.3 Vibrating Mesh Nebulizers
Vibrating mesh (or plate) nebulizers employ a small plate containing ≅1,000 very small cone-shaped holes through which a liquid is forced by vibrating the plate at very high frequencies over a thin layer of liquid. As the liquid is forced through the holes in the vibrating plate, it is aerosolized. These nebulizers demonstrate increased efficiency of drug delivery to the respiratory tract compared to some pneumatic jet nebulizers (Dubus et al. 2005; Dhand 2002; Skaria and Smaldone 2010; Ari et al. 2010a, b). However, there are different design approaches between brands of these types of nebulizers (Ari et al. 2009), and as with pneumatic nebulizers, there are most probably significant performance differences between brands.
Vibrating mesh nebulizers also have the added advantage of not generating any additional flow, which pneumatic nebulizers do. This is important if the nebulizer is placed in-line in the circuit of some types of ventilators. Many of the latest generation of critical care ventilators measure flow going into and out of the ventilator circuit. These flow measurements are used in feedback loops by the ventilator for, among other things, disconnection alarm management. When a pneumatic nebulizer is placed in-line, the flow used to operate the nebulizer is added into the ventilator circuit, which can potentially affect efficiency of some alarms and the accuracy of some flow measurements. In some older models of ventilators, this added flow can inadvertently increase tidal volume by relatively large amounts when very low tidal volume settings are used (Salyer et al. 1990).
One disadvantage of the vibrating mesh nebulizers is that they are more costly than the typical disposable pneumatic nebulizer or MDI with spacer/holding chamber. However, such costs can be easily justified when viewed in the light of the overall cost of being on a ventilator and in an intensive care unit. The use of more effective aerosol-generating technology might well shorten length ventilation and thus length of stay in the intensive care unit, although this has yet to be proven.
30.1.3.4 Metered-Dose Inhalers
Metered-dose inhalers are small canisters filled with a pressurized propellant and medication. When the MDI is actuated, the propellant and medication mix and are rapidly expelled from the MDI in a plume of aerosol (Fig. 30.4) (Newman 2005). The velocity of this plume of aerosol-laden gas makes the successful use of MDIs very technique dependent. The actuation of the MDI needs to be synchronized with the inspiratory cycle. If the output of an MDI is pointed directly into the mouth, much of the aerosol will be deposited in the mouth and pharynx because the high initial velocity of the plume causes inertial impaction of particles in the mouth. This phenomenon also occurs if the MDI is actuated directly into a ventilator circuit by use of certain types of adapters, with much of the drug being lost in the circuit and the connectors.
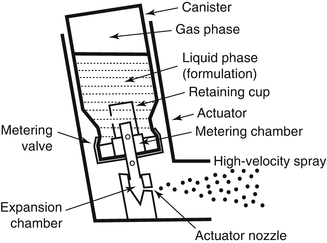
Fig. 30.4
Schematic diagram of metered-dose inhaler (Used with permission from Newman (2005))
One way to overcome this is the use of certain types of adapters, spacers, or holding chambers to improve the interface of the MDI with the patient’s ventilator circuit/airway. Types of adapters include elbow adapters, unidirectional or bidirectional in-line adapters, spacers, and holding chambers. The elbow adapters typically connect to the ETT, whereas the in-line adapters, spacers, and chamber adapters may be placed in the inspiratory limb of the ventilator circuit or connected directly to the artificial airway.
The MDI spacer is a quasi-cylindrical reservoir that holds several 100 mm of gas. The MDI plume mixes with the gas in the spacer and slows the velocity of the plum while the aerosol remains suspended so it can be inhaled. This significantly reduces inertial impaction.
A holding chamber is a spacer that is designed with valves in such a fashion to separate inspiratory and expiratory flow. This valved configuration allows infants and children to be given several subsequent breaths while connected to the holding chamber, thereby increasing the likelihood that more of the emitted dose of the MDI will be deposited in the lungs. These were developed in part because of concerns that the spacer could act as mechanical dead space if the patient was allowed to exhale into the spacer and rebreathe the gas in the spacer. However, it has been shown in laboratory modeling that very little CO2 is rebreathed during drug administration using an MDI with spacer (Lugo et al. 2000). Nevertheless, some clinicians still feel that limiting the time the spacer is in the circuit is necessary to avoid CO2 rebreathing, but this technique has been shown to reduce the amount of drug delivered to the patient (Lugo and Ballard 2004).
But not all spacers and valved holding chambers are created equal either. Most brands of MDI spacers or holding chambers are manufactured from materials that acquire an electrostatic charge on the inner surface. This charge tends to attract suspended aerosol particles which deposit on the inner walls of the spacer, significantly reducing bioavailability of the drug (Wildhaber et al. 1996). This effect can be diminished by rinsing the inside of the chamber with soap and water. However, this is not as effective as using spacers and holding chambers made from non-electrostatic materials, which allow for a significantly larger portion of the emitted dose to exit the spacer (Rau et al. 2006; Nair et al. 2009). Figure 30.5 illustrates differences in the amount of emitted dose from an MDI that is used with holding chambers that were made from electrostatic versus non-electrostatic materials. The PARI Vortex (PARI Inc) and AeroChamber MAX (Monaghan Medical) products were both made of non-electrostatic materials. The use of a spacer/holding chamber with the MDI during mechanical ventilation results in a four to six-fold increase in drug delivery when compared with an elbow adapter or unidirectional in-line adapter (Dhand 2005; Bishop et al. 1990; Rau et al. 1992; Fuller et al. 1994; Diot et al. 1995). While MDIs are better than pneumatic nebulizers at delivering inhaled medication to ventilated patients, (Hess 2002; Dhand and Guntur 2008; Lugo et al. 2001) this is only true if the devices are used with the appropriate interface with the ventilator circuit.
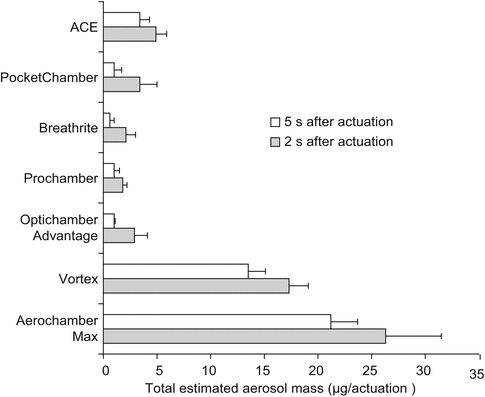
Fig. 30.5
Results of a study of the effect of using non-electrostatic material to construct spacers and holding chambers. Displayed from several brands of spacers. The Vortex and AeroChamber products are both constructed of non-electrostatic materials. This effect was scene both before and after rinsing of the chambers (Adapted from Rau et al. (2006))
Conventional wisdom in the recent past suggested that MDIs with spacers or valved holding chambers were more expensive than disposable pneumatic nebulizers. We demonstrated that when sophisticated costing models were used that analyzed the total cost of aerosolized medication administration, including labor, MDIs with valved holding chambers were less costly than pneumatic nebulizers in a pediatric population (Salyer et al. 2008).
30.1.3.5 Location of the Aerosol Generator
Figure 30.6 illustrates various options for location of an aerosol-generating device in a ventilator circuit. Device location has been demonstrated to have a large effect on drug delivery. In a series of well-designed experiments, Ari and colleagues (Ari et al. 2010b) have described the effects of location, nebulizer type, humidification, and bias flow. Figure 30.7 illustrates some of the results of their work. They demonstrated that by variously combining these factors, drug delivery past the endotracheal tube could be increased from 2.5 to 30 % of the emitted dose. Generally, the optimum location is dependent on the type of aerosol-generating device. For MDIs with spacer, the best location was between the ventilator circuit and endotracheal tube. For pneumatic (jet) nebulizers, the optimal location appears to be in the inspiratory limb of the ventilator circuit, a short distance (≅15 cm) from the Y-piece. For vibrating mesh nebulizers, the optimal location was also in the inspiratory limb of the circuit.
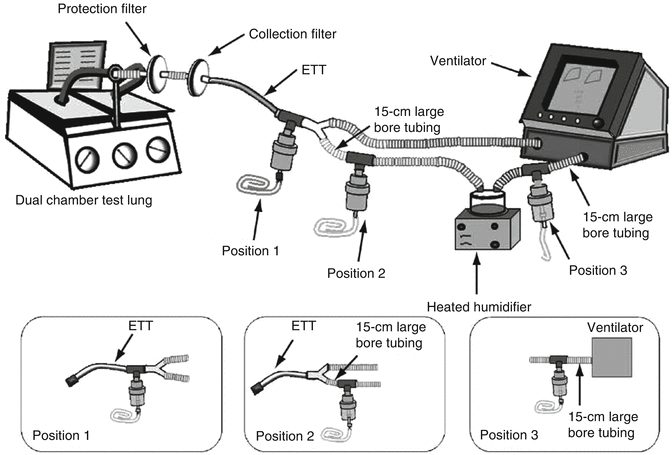
Fig. 30.6
Schematic representation of typical options for placing aerosol-generating devices in the ventilator circuit during mechanical ventilation, including dual-chamber test lung, filters, endotracheal tube, and ventilator circuit, with and without heated wires. Three aerosol generator positions are shown (Used with permission from Ari et al. (2010b))
Another option is to ventilate the patient with a manual resuscitator that is connected to the MDI-holding chamber assembly which in turn is connected to the ventilator. However, it has repeatedly shown that ventilation can be highly variable during neonatal or pediatric manual ventilation and that this variability is dependent in part on the type of resuscitator used (Salyer 2009). Thus, manual ventilation introduces more potential variation into a procedure that already demonstrates high variability in efficacy and is probably not generally recommended.
30.1.3.6 Humidification of Inhaled Gas
It has been known for some time that the aerosolized drug delivery is adversely affected by the humidification of the carrier gas (Lange and Finlay 2000; Fink et al. 1999; Miller et al. 2003). Ari and her colleagues added to this understanding, showing that humidified carrier gas reduces the efficacy of aerosol delivery and that this effect is more pronounced the longer the aerosol resides in the humidified gas stream for nebulizers. This phenomenon occurs because some types of aerosol particles are hydrophilic, which means that in the presences of higher relative humidity, water comes out of vapor phase and attaches to the outside of the particle, increasing its size. Thus there are a higher proportion of larger particles and more inertial impaction of aerosol particles in parts of the delivery system and airways where they cannot be therapeutic.(Balásházy et al. 2007)
30.1.3.7 Ventilator Settings
Theoretically, higher flow rates should result in more turbulent movement of gas through the airways. Through the phenomena of coalescence, at higher flow rates there will be more collisions of aerosol particles, resulting in the formation of larger particles. Loss of aerosol through inertial impaction will also be increased as flow rates increases because particles will have more linear momentum as the mover through the tortuous path of the airways. And larger tidal volumes should also enhance drug delivery due to increased dilation of the airways during the positive pressure phase of the inspiratory cycle. An inspiratory pause ought to be efficacious as it should allow for more deposition from gravitational sedimentation.
However, research findings on the effect of ventilator settings are divergent. Ari et al. demonstrated in adult and pediatric lung models that increasing bias flow decreases drug delivery (see Fig. 30.8) (Ari et al. 2010a). Others have demonstrated that increasing tidal volume, lowering flow rates, lengthening inspiratory times, and synchronizing devices with the patients’ inspiratory effort improve drug delivery (Fink et al. 1996, 1999; O’Riordan et al. 1992; Dolovich 2000; Dhand 2088). Again, most of this work has been done using in vitro lung models.
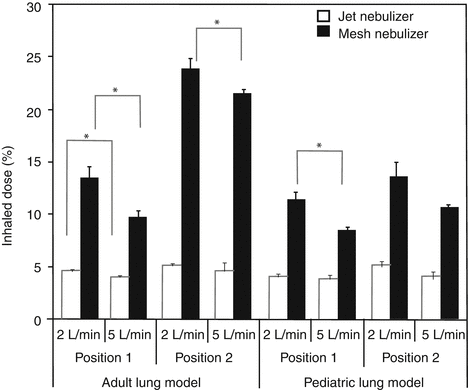
Fig. 30.8
The effect of increasing the bias flow on the delivery of aerosolized medications past the tip of the endotracheal tube in an adult and pediatric lung model. Position 1 is in the inspiratory limb ≅15 cm from the Y-piece, while position 2 is in between the outlet of the ventilator and the heated humidifier which was operated at 35 °C. Adult V T = 500 mL, pediatric V T = 100 mL (Used with permission from Ari et al. (2010a)). Asterisk denotes statistical significance
But Mouloudi et al. were unable to detect any difference in the clinical response to inhaled bronchodilators delivered via MDI with spacer to ventilated patients when flow rates were decreased nor when flow patterns were altered from decelerating to continuous inspiratory flow profiles nor when an inspiratory pause was introduced nor when tidal volume was increased by 4 ml/kg (Mouloudi et al. 1998, 1999; 2000; Malliotakis et al. 2007). Garner and colleagues used a neonatal ventilation model to show that albuterol delivery was not different in any of the three different modes and when large increases in spontaneous respiratory rate were introduced (Garner et al. 2002). The divergence of findings in the effect of ventilator settings on drug delivery might well be related to the wide variety of aerosol-generating devices tested and a lack of standardized device location and use of humidification.
This divergence notwithstanding, the majority of the published literature is in support of the idea that aerosolized drug delivery is indeed affected by alterations in the ventilator settings as described above. It seems prudent and reasonable to try to optimize drug delivery by using ventilator settings that serve the dual purpose of meeting the patients’ physiologic demands and improve the amount of drug delivery. Especially since delivery of aerosolized drugs is so inefficient, even under optimal conditions.
30.2 Specific Inhalational Treatment
30.2.1 Bronchodilator Therapy
John Salyer8
(8)
Department of Respiratory Care, Seattle Children’s Hospital Foundation, Research Institute, 4800 Sand Point Way NE, Seattle, WA 98105, USA
30.2.1.1 Efficacy and Utilization of Inhaled Bronchodilators
It has been suggested by some that there is little evidence that bronchodilator therapy has any influence on patient outcomes related to pulmonary disease during mechanical ventilation (Dhand 2007; Chang et al. 2007). The mostly widely used inhaled bronchodilators during mechanical ventilation are β2 agonists like albuterol (salbutamol). My observations indicate that the drug is overutilized in ventilated patients and this has also been reported in the literature (Dhand 2088). Chang and colleagues reported that 36 % mechanically ventilated patients who do not have clinical evidence of obstructive lung disease received inhaled bronchodilators during their ventilator course (Chang et al. 2007). I have also observed that many patients do not appear to have any “apparent” response to the medication. Although the most common clinical goal for aerosolized β2 agonists during mechanical ventilation is relief of bronchospasm, other indications for this drug and route have been reported.
Benefits of inhaled β2 agonists in mechanically ventilated patients have been reported to include:
-
Relief of bronchospasm: Airway resistance (R aw) will decrease as bronchospasm is relieved in ventilated patients. Technically, the measurement of R aw (and lung compliance) requires the insertion of an esophageal balloon to facilitate measurement of esophageal pressure which is used as a surrogate for intrapleural pressure. Since this is rarely done in most clinical scenarios, resistance of the total respiratory system (R RS) is used as a surrogate. This value is typically displayed as cmH2O/L/s and is calculated by most contemporary critical care ventilators. Owing to imprecision and normal variability of these types of biological measurements, a decrease of >15 % is usually required to be confident there has been a response to bronchodilators (Torres et al. 1997). And care should be taken to look at this displayed value on the ventilator for several breaths to make sure there is reproducibility, since erratic spontaneous breathing patterns and ventilator dyssynchrony are prevalent in infants (Mouloudi et al. 1998; Paetow et al. 1999; Kapasi et al. 2001). If the ventilator used does not calculate resistance, peak airway pressure decreases can be a surrogate for response to bronchodilators, but only if the patient is in a volume-controlled, decelerating flow mode of ventilation. Obviously, peak airway pressure will remain constant in pressure-controlled modes. And there can be other causes for decreasing airway pressures including removal of retained secretions. This is another reason why it is a good idea to do pulmonary toilet before you give an inhaled bronchodilator. Another way to evaluate response to bronchodilators is to evaluate the appearance of the flow volume loops. Holt and colleagues illustrated this in Fig. 30.9 (Holt et al. 1995). Compliance of the respiratory system (C RS) has also been shown to improve significantly after bronchodilator administration in ventilated infants (Rotschild et al. 1989; Denjean et al. 1992; Sivakumar et al. 1999). Again, changes of >15 % is necessary to have confidence of a real clinical response.Fig. 30.9Flow volume loops during mechanical breaths of an infant who had a positive response to aerosolized bronchodilators. (a) Before treatment and (b) 20 min after treatment during mechanical breaths (Used with permission from Holt et al. (1995))
-
Improved mucociliary clearance: Mucociliary clearance has been reported to be profoundly diminished in patients who require mechanical ventilation (Konrad et al. 1994, 1995; Dolovich et al. 1998). Inhaled β2 agonists have been shown to improve mucociliary clearance (Bennett 2002; Houtmeyers et al. 1999; Foster et al. 1976; Gatto 1993). The effect seems to be more enhanced at higher doses and for prolonged therapy (Dolovich 2000). This improvement in mucous transport is caused by an increase in mucociliary beat frequency. However, a link between increased ciliary beat frequency and improved pulmonary outcomes in patients receiving mechanical ventilation has not yet been established, though some investigators have tried and failed to find an effect on outcomes (O’Riordan et al. 2006).
-
Treatment of hyperkalemia: Elevated serum levels of potassium are a common complication of intensive care (Buckley et al. 2010). Inhaled β2 agonists are known to induce hypokalemia (Burgess et al. 1989; Allon and Copkney 1990; Habashy et al. 2003; Hung et al. 1999). In one study of ventilated neonates receiving inhaled albuterol, 28–36 % of these very low-birth-weight infants developed hypokalemia (Mhanna et al. 2009). This effect appears to be prevalent only after many repeated doses since clinically significant reductions in serum potassium levels were not seen when six doses of inhaled β2 agonists were given over a period of 3 h in one study of pediatric asthma patients (Kaashmiri et al. 2010).
-
Improvement in pulmonary mechanics: As stated above, inhaled β2 agonists have been shown to reduce R RS C RS in ventilated children. In addition, inhaled β2 agonists have been reported to (1) reduce plateau pressures and reduce airflow resistance in ARDS, (Wright et al. 1994); (Gay et al. 1987) (2) lower intrinsic PEEP (Morina et al. 1997), (3) and decrease work of breathing (Mancebo et al. 1991).
It is widely held among respiratory therapists that inhaled bronchodilators in ventilated patients are overutilized, which is probably true, but there is no published literature on this subject. In general, the utilization of respiratory therapy procedures is almost always reduced when standardized clinical protocols are introduced. There is no reason to presume that the utilization of inhaled bronchodilators to mechanically ventilated infants and children would not also benefit from protocol standardization that would result from the use of systematic protocols (Stoller 2001).
30.2.2 Inhaled Selective Pulmonary Vasodilators
Peter Dahlem9
(9)
Department of Pediatrics, Medical Center of Coburg, Ketschendorferstr. 33, Coburg, 96450, Germany
Educational Aims
To understand that:
-
Hypoxemia in ALI is caused by ventilation/perfusion mismatch.
Stay updated, free articles. Join our Telegram channel
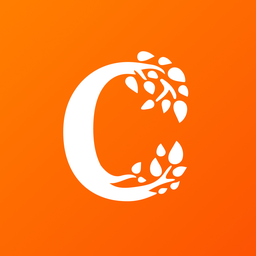
Full access? Get Clinical Tree
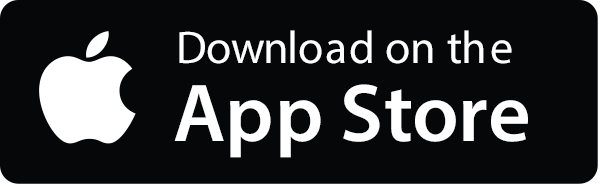
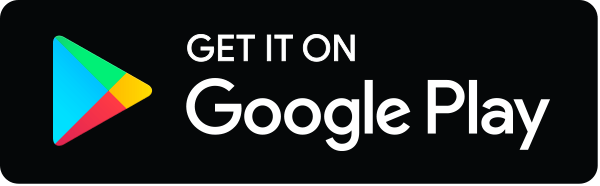