Drug Metabolism and Disposition in Infants and Children
Anders Rane
The new regulatory framework for development of drugs for children has promoted the interest in pediatric pharmacology and left behind the traditional pediatric pharmacotherapeutics based on cautious and conservative empiricism. Not only economical incentives but also new guidelines on ethics for pediatric age groups have contributed to this positive development. Several investigations in recent years have evidenced the great dearth of documentation on drugs for children and the extensive off-label use of drugs for pediatric age groups. This situation is metabolised by enzymes with genetic polymorphisms.
The aim of all treatment with drugs is to obtain a specific pharmacologic response with a minimum risk of adverse effects. This is best achieved by selection of well-documented drugs based on comprehensive knowledge about drug behavior and sensitivity in the target group of patients. Due consideration of the large interindividual variability in drug response (pharmacodynamics) and disposition (pharmacokinetics) as well as of disease state and other patient features is required. For most drugs, interindividual variability in drug response is less pronounced than the variability in drug disposition. It is also evident that the age-dependent variation in drug kinetics is larger than the genetic variation for most drugs, except for drugs.
Therapeutic disasters with sulfonamides (1) and chloramphenicol (2) involving toxic effects in the newborn after administration of the same body-weight-related doses as to adult patients are examples of the clinical consequences of altered drug disposition in the young. These events have contributed to the dogma that the infant has an augmented response to drugs throughout development. We know that this is not always the case.
Age-related changes in drug disposition are related to maturational increases in liver and kidney function and variations in plasma protein binding, tissue distribution, and gastrointestinal absorption. Other physiologic parameters also contribute to the rapid changes. Development of drug analytical techniques has been a perequisite for detailed pharmacokinetic studies. However, there is a dearth of understanding and knowledge about the concentration-effect relationships in infants and children. These aspects of developmental pharmacology are important areas for future research because it is not possible to extrapolate these relationships from adult patients to children.
This chapter discusses how the development of certain constitutional features may affect drug disposition in infants and children. To illustrate this, several examples from important pharmacotherapeutic areas are presented.
Drug Metabolism as Reflected by Clinical Pharmacokinetic Data
Concepts
Elimination of drugs and other xenobiotics terminates their effects. Elimination involves various excretory pathways with or without a preceding chemical alteration of the drug molecule, enzymatically or nonenzymatically. The elimination processes may be seen as part of the body’s defense mechanism. Without these processes, drug accumulation and toxicity would ensue. The sum of all elimination processes is equal to the total-body intravenous (IV) clearance (ClIV) of the drug. This, by definition, is the volume of blood or plasma that is irreversibly cleared of drug per unit time. If an IV dose D of the drug is administered to the patient and the area under the plasma concentration-versus-time curve (AUCIV) is assessed, the ClIV can be calculated from

The ClIV reflects the sum of the different elimination routes. Thus, ClIV may be the sum of hepatic (ClH) and renal (ClR) clearance as well as other clearance mechanisms. If the drug is administered orally, the apparent oral clearance (ClO) (consider the fraction F of the oral dose that reaches the systemic circulation) is:

It follows that 1 – F is the fraction of the oral dose that is metabolized or otherwise eliminated in the liver and/or gut wall during passage from the gut to the systemic circulation. This phenomenon is called first-pass elimination. If all the drug in the gut reaches the portal vein, then

where E is the hepatic extraction ratio. This is the proportion of the dose that is eliminated during one passage through the liver. The endothelial cells lining the villi harbor drug-metabolizing enzymes that may contribute to the first-pass elimination. In addition, many drugs are acted on by ATP-binding cassette (ABC) transporter proteins or organic anion-transporting polypeptides (OATP) in the endothelial cells and pumped out of the cells back into the gut lumen. If only part of the oral dose reaches the portal vein, the deficient absorption or uptake must be corrected for in the calculations of E and F.
The relationship between ClH, the liver blood flow Q, and the total intrinsic hepatic clearance Cli is defined by the perfusion-limited clearance model (3), (4) for drug clearance in the liver. This relation is defined as

The intrinsic clearance is defined as the maximum capacity of the liver (or any other organ) to remove drug from the blood in the absence of flow limitations. From Equation (4) it is obvious that changes in liver blood flow will preferentially affect the clearance of drugs with high values of Cli, which may be observed as changes in plasma half-life t1/2. In contrast, changes in enzyme activity will affect the t1/2 only of drugs with low values of Cli. In addition, such changes will affect the AUC both after oral and IV administration such that the AUC is decreased when the enzyme activity is enhanced.
The drug clearance from an organ is also dependent on drug binding in the blood by modification of Equation (4):

where fB denotes the unbound fraction in blood and Cli′ is the intrinsic hepatic clearance of unbound drug. If Cli′ is high, drug binding in blood has little importance for the ClH. In contrast, drug binding has a limiting (restrictive) influence on ClH if the value of Cli′ is low (5). As a corollary, hepatic drug extraction from the blood is denoted as nonrestrictive or restrictive, respectively. The derivation of the hepatic clearance is important since the determinants are subject to age-dependent development.
Clinical Data
Estimation of metabolic clearance is of interest in pediatric pharmacology since many enzymatic fractions develop slowly in neonatal life. As the hepatic blood flow varies, in vitro estimates such as intrinsic clearance (Cli = Vmax/Km) are not very useful. Therefore, in vivo methods for estimating Cli are preferred. By definition, ClO is equivalent to the intrinsic hepatic clearance Cli of the drug, provided the drug is eliminated only by liver metabolism and is completely bioavailable to the portal vein. However, very few drugs fulfill these criteria, and in children, no data on the apparent oral clearance of such drugs seem to exist.
The plasma half-life t1/2 may serve as an estimate of the hepatic drug-metabolizing activity only under certain criteria. The t1/2 gives no information about the efficiency of drug elimination processes even though it provides a rough estimation of duration of effect. If the drug is solely eliminated by hepatic metabolism, that is, if systemic clearance Cls is equal to ClH, then

This relationship demonstrates that t1/2 depends on ClH as well as on the apparent volume of distribution Vd of the drug.
As is evident from Equation (5), hepatic clearance ClH is a function not only of enzyme activity (Cli) and drug binding in blood (fB) but also of blood flow (Q). Therefore, to interpret kinetics data from systemic administration of drugs at different stages of development, it is important to differentiate between low-extraction and high-extraction drugs. A number of drugs have been classified according to this system on the basis of adult human pharmacokinetic data (Table 4.1).
Drug distribution is affected by a variety of physiologic factors, including vascular tissue perfusion, body composition, plasma protein binding, and tissue binding. Because the Vd may be subject to developmental changes, caution must be exercised in the interpretation of data on t1/2 in infants and children. However, if we make a reasonable assumption that Vd is constant, then t1/2 after an IV administration of the drug is virtually proportional to the hepatic enzyme activity (for low-extraction drugs), the hepatic blood flow (for high-extraction drugs), or both (for drugs with intermediate values of Cli).
The steady-state concentration Css of a drug administered orally and metabolized only by the liver is given by the following equation:

where Δ denotes the dosing interval and F is the drug bioavailability. Rearranging this equation gives

This relationship shows that the only biologic determinants of Css of a drug that is given orally and solely metabolized by the liver are the Cli′ and the binding, irrespective of the extraction ratio (3), (6). In this context, any discussion about blood flow is superfluous.
Table 4.1 Comparison of Half-Lives in Newborns and Adults of Drugs with Low or High Hepatic Extraction | ||||||||||||||||||||||||||||||||||||||||||||||||||||||||||||||||||||||||||
---|---|---|---|---|---|---|---|---|---|---|---|---|---|---|---|---|---|---|---|---|---|---|---|---|---|---|---|---|---|---|---|---|---|---|---|---|---|---|---|---|---|---|---|---|---|---|---|---|---|---|---|---|---|---|---|---|---|---|---|---|---|---|---|---|---|---|---|---|---|---|---|---|---|---|
|
Most pharmacokinetic data in children were obtained through classical studies requiring “rich” data from the individual patient. Modern therapeutic drug monitoring, improvements in drug assay methodology, and large-scale therapeutic drug monitoring in children have promoted the introduction of population pharmacokinetic modeling methods to achieve optimization and safety of pediatric drug treatment. Population pharmacokinetics is particularly useful in pediatric therapy since it is possible to use and analyze sparse data from a large number of subjects. The mixed-effects model includes fixed effects as well as random effects on variability between subjects. Data capture from a large number of subjects and application of statistical programs such as nonlinear mixed-effects model have yielded a lot of clinically useful pharmacokinetic information in children (7) and been applied in studies of, for example, pantoprazol (8), levetiracetam (9), and other drugs in children. Population modeling can also be used to explore different components of the developmental variation in pharmacokinetics such as maturation of clearance pathways, receptor functions, and so forth. This approach will be increasingly used in investigations of drugs for children.
Low-Clearance Drugs
Among the drugs that belong to this group are antiepileptics and certain benzodiazepines. They are frequently used in infants, and their t1/2 values are predominantly dependent on the drug-metabolizing enzyme activity. The Css of these agents is determined by the hepatic enzyme activity and by drug binding in blood according to Equation (8). Inasmuch as the values of fB and Vd are not age dependent, the t1/2 and Css may serve as rough estimates of the capacity to metabolize the drug. Some of the drugs of this group that have been studied in newborns and adults are listed in Table 4.1.
The t1/2 of carbamazepine and phenytoin in newborn infants of epileptic mothers treated with these agents during pregnancy is similar to the t1/2 in adults (10), (11). This is probably due to intrauterine induction of the drug metabolism. As judged from the t1/2 values, the capacity to metabolize phenytoin in the newborn seems to be well developed at birth, as the Vd of phenytoin does not change from the neonatal to the adult stage (12).
The Vmax for oxidation of phenytoin is higher in children than in adults (13), and the capacity to oxidize phenytoin decreases with age (14). The recommendation of higher weight-related doses of these antiepileptics in children compared with adults is therefore logical (see later discussion).
Theophylline and caffeine have comparatively long plasma half-lives in the neonatal period (Table 4.1), whereas in adults the corresponding values are only 3.8 to 8 (15,16) and 4 hours (17), respectively. This difference (17,18,19) is consistent with the extremely low and undeveloped fetal hepatic in vitro activity of cytochrome P4501A (20), which is the major catalyst of the oxidation of theophylline and caffeine (21), (22).
High-Clearance Drugs
The t1/2 of drugs belonging to this group is dependent to a large extent on hepatic blood flow, whereas variation in enzyme activity has less influence on the elimination of an IV dose. However, enzyme activity does determine the AUC and Css values after single and multiple oral administration, respectively. Although the use of these drugs is limited in children, some kinetic data for these agents have been published (Table 4.1).
Table 4.2 lists the half-lives of some drugs unclassified with respect to their clearance values. The general impression is that the half-life of most drugs in the neonate is longer than in the adult patient. This strongly suggests that most investigated drugs have a lower metabolic clearance in neonates and infants than in the adult (as judged from the values of the t1/2). The rate of maturation of this capacity varies from drug to drug. As a corollary, attempts to predict the drug-metabolizing activity in children from adult data are bound to fail.
Table 4.2 Comparison of Plasma Half-Lives in Newborns and Adults of Some Drugs that are Unclassified with Respect to Hepatic Clearance | ||||||||||||||||||||||||||||||||||||||||||||||||||
---|---|---|---|---|---|---|---|---|---|---|---|---|---|---|---|---|---|---|---|---|---|---|---|---|---|---|---|---|---|---|---|---|---|---|---|---|---|---|---|---|---|---|---|---|---|---|---|---|---|---|
|
Drug Binding to Plasma Proteins
Because a drug’s pharmacologic effects and side effects are related to the unbound fraction of drug in blood fB, it is relevant to know how fB varies in different age groups. Routine analyses of drugs in plasma generally account only for the total concentration. As long as the drug binding does not vary substantially between individuals in the same age group, specific analysis of the unbound fraction is generally not warranted. However, such analysis may have a clinical value in special patients, for example, if the plasma protein level is perturbed by compromised kidney function or liver disease.
Most investigated drugs are less bound in cord/infant plasma than in adult plasma (Table 4.3). This may have several explanations. The plasma protein may be qualitatively or quantitatively different at this age. The albumin concentration in neonates appears to be equivalent to the adult concentration (23), but α1-acid glycoprotein concentrations are lower (24). In addition, hypoproteinemia is frequently observed in premature infants (23). The possible influence of competing ligands on drug binding has been discussed for bilirubin (25) and free fatty acids (26). Such factors may be the reason for the lower serum protein binding of many drugs, such as clonazepam (27). For clonazepam, adults have a lower binding capacity but a higher affinity to serum proteins than children.
As pointed out earlier, the clearance term, Equation (5), is affected by drug binding in blood and plasma. For low-clearance drugs, the hepatic elimination is usually restrictive and the systemic clearance of total drug will depend on binding. The t1/2 is prolonged when binding increases. The concentration of unbound drug is essentially unchanged. On the other hand, for drugs with a high clearance, a decreased binding leads to a higher unbound concentration and more pronounced pharmacologic effects (4).
Table 4.3 Protein Binding of Some Drugs in Cord Plasma in Relation to Adult Plasma | ||||||||||||||||||||||||||||||||||||||||||||||||||||||||||||||
---|---|---|---|---|---|---|---|---|---|---|---|---|---|---|---|---|---|---|---|---|---|---|---|---|---|---|---|---|---|---|---|---|---|---|---|---|---|---|---|---|---|---|---|---|---|---|---|---|---|---|---|---|---|---|---|---|---|---|---|---|---|---|
|
The differential effect of altered drug binding on the total plasma concentration of high-clearance and low-clearance drugs needs consideration in studies of drug concentration-effect relationships.
The steady-state concentration Css of phenytoin that is attained for a given dose per kilogram body weight is considerably lower in infants than in adults, even though the infant dose is twice as high on a body-weight basis (25), (28). This may be due, in part, to binding alterations, because a lower binding yields a lower total plasma concentration. However, it is believed that a higher metabolic rate at this age contributes substantially to the low Css in these infants. The issue of the appropriate therapeutic concentration of the drug in infants is one of great interest. It is conceivable that the higher fB compensates partially for the lower total Css values that are attained (25).
Bioavailability: Drug Absorption and Transporter Proteins
The drug absorption process at various ages is not well understood. The gastric pH is alkaline at birth but falls to pH 1 to 3 within 1 or 2 days (29). Adult levels of gastric acid secretion are reached at ages 5 to 12 years (for a review, see reference 27). Although the nonionic diffusion principle would favor absorption of acidic drugs in the stomach, a substantial part of the important absorption takes place in the duodenum. The absorption of penicillin G and semisynthetic penicillins is higher in newborn infants than in adults (29). This may be explained, in part, by the higher pH in newborn infants.
The motility of the gut and the gastric emptying time have been reported to be delayed in newborn infants (30). Although this may affect the absorption-time profile rather than the degree of absorption, it has been proposed as an explanation for the erratic absorption of certain sustained-release theophylline formulations in children (31). There are data to support this assumption. Heimann (32) studied the bioavailability of sulfonamides, digoxin, and some other drugs in infants and children. Although the rate of absorption was significantly lower in neonates, the amount of drug absorbed was not correlated with age.
The fetal gastrointestinal tract is rapidly colonized by bacteria after birth. The level of the gastrointestinal microorganism flora is related to bile acid deconjugation and β-glucuronidase activity, both of which are significantly higher in neonates than in adults (33). Other intestinal enzymes that may affect the drug absorption and are subject to age-dependent alterations include lipase and α-amylase (34), both of which are low in the neonatal period.
Many drugs are substrates of the ABC-transporter system, of which p-glycoprotein is the best known and most investigated member. These protein pumps transport drugs across membranes, for example, in the blood-brain barrier and placenta, where they can be protective against foreign compounds in the (maternal) circulation, or in the endothelial cells of the gastrointestinal tract, where they can pump drugs back into the gut lumen. The function of these pumps affects the oral bioavailability, F, as do the drug-metabolizing enzymes that are co-located with the p-glycoprotein in the endothelium. Another large family of influx transporters is the organic anion transporting proteins (OATP) which have a role in the transport of several drugs, e.g. statins, across many membranes in the body. Little is known about the development of these transporter systems during maturation.
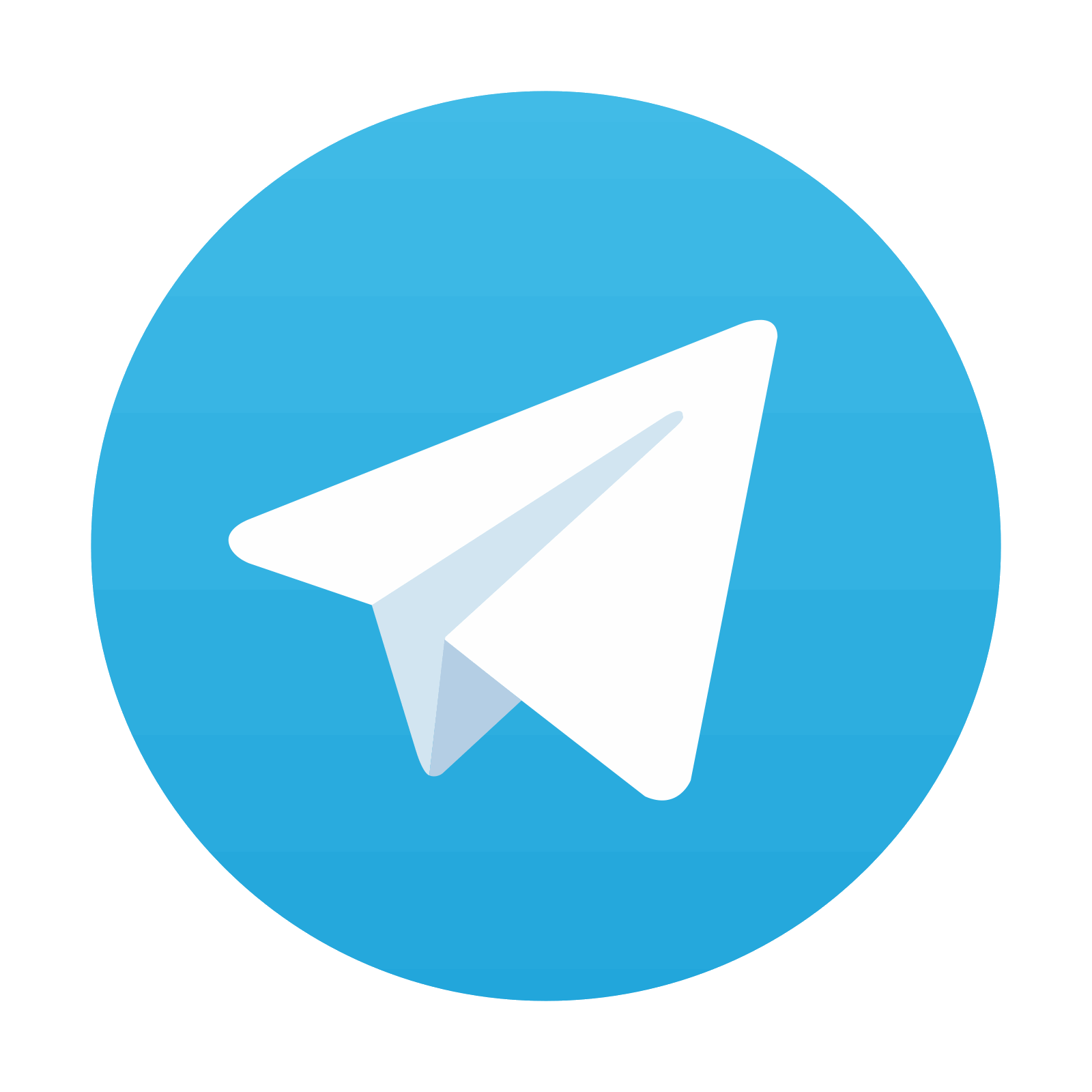
Stay updated, free articles. Join our Telegram channel
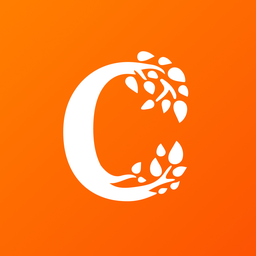
Full access? Get Clinical Tree
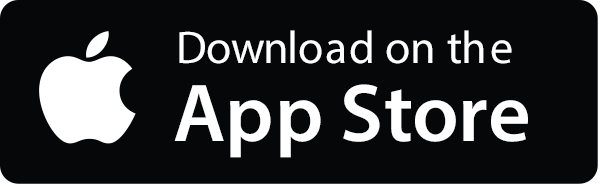
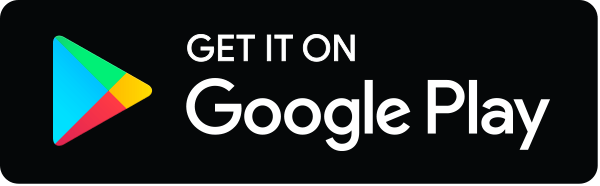