-
Chapter Contents
-
Part 1: Renal function and renal disease in the newborn 927
-
Part 2: Urology 939
Renal function and renal disease in the newborn
- Neena Modi
Morphological development of the kidney
The kidney develops from the caudal segment of the nephrogenic ridge, the metanephros , a population of mesodermal cells that condense around the tip of the ureteric bud from about 5 weeks’ gestation. Nephron formation is induced as the ureteric bud branches and rebranches, forming first the major, then the minor calices, and finally the arborising system of collecting ducts. Nephrogenesis proceeds centrifugally with the juxtamedullary nephrons that lie deepest in the cortex developing first, and those in the superficial (subcapsular) cortex last. Urine production begins at 8–10 weeks’ gestation. The total complement of around 1 million nephrons is complete by 36 weeks. Nephrons that die are not replaced and subsequent increase in renal mass is due to tubular growth.
Assessing renal function
Glomerular filtration rate
Although widely described as ‘low’ in the newborn, glomerular filtration rate (GFR) is adequate for need. Formal measurement is not usually made and the serum or plasma creatinine (Pcr) is used as a proxy. Creatinine is derived from the turnover of phosphocreatine in muscle and excreted in the urine. The Pcr at birth reflects the maternal concentration. Subsequently this changes at a rate based on the balance between creatinine production rate, dependent on muscle mass ( ), and clearance, dependent on GFR, that varies with postconceptional age ( Fig. 35.1 ). The wide range in Pcr against postnatal age is due predominantly to the large variation that exists in weight for postconceptional age.

Pcr initially falls rapidly as the maternally derived load is excreted, then more gradually ( ). In term newborns, the Pcr at birth is typically 70–90 µmol/l (about 1 mg/dl), falling to about 30 µmol/l (0.3 mg/dl), with a range of 15–40 µmol/l (0.17–0.45 mg/dl), by 1 week of age and remaining at this level for the rest of the first month. A single measure of Pcr provides no more than a crude estimate of renal function. Observing the change over days is of more help. In the neonate, a sustained rise in Pcr, or a failure to fall, is often indicative of reduced GFR ( Fig. 35.2 ). Blood urea is not recommended for assessment of renal function as it is influenced by numerous non-renal factors such as an elevated level from sequestered blood in the gastrointestinal tract.

Tubular function
Amino acids
In term neonates mild aminoaciduria is present, particularly affecting glycine, imino acids (proline and hydroxyproline), dibasic amino acids and taurine. Fractional reabsorption of other amino acids is relatively complete ( ).
Glucose
Mean urinary glucose concentration in the term newborn is slightly higher than in older children and adults (mean values 0.83 mmol/l (15 mg/dl) versus 0.33 mmol/l (6 mg/dl)) ( ). More marked glycosuria in a term infant cannot be attributed to physiological immaturity and should be investigated. Even moderate hyperglycaemia in the presence of a low threshold leads to substantial glycosuria and osmotic diuresis. It is essential that blood glucose is closely monitored in infants receiving intravenous fluids and parenteral nutrition.
Phosphate
Normal plasma phosphate concentration is higher in the fetus and healthy newborn infant than in older subjects. This is associated with a renal tubular phosphate threshold (plasma phosphate concentration above which phosphate appears in the urine) that is higher than that in adults ( ), reflecting the need for phosphate retention in the rapidly growing infant, mainly to support skeletal growth. Since human milk contains little or no excess phosphate over the dietary needs of the infant, urinary phosphate excretion is normally low. Preterm infants are vulnerable to metabolic bone disease secondary to phosphate deficiency. Phosphaturia in the presence of normal or low plasma phosphate concentrations should be investigated.
Sodium
Healthy term infants are able to produce virtually sodium-free urine but the capacity to excrete a sodium load is less well developed than in older individuals ( ). The preterm newborn’s ability to conserve and excrete sodium is compromised, and hence these babies are vulnerable to both sodium depletion and overload. In adults, approximately 80–90% of filtered sodium is reabsorbed in the proximal tubule ( Fig. 35.3 ) ( ) and in the thick ascending limb of the loop of Henle. Unabsorbed sodium passes into the distal tubule and collecting duct where absorption occurs in exchange for potassium and hydrogen. Distal tubular sodium reabsorption is regulated by the renin–angiotensin–aldosterone system (RAAS). In neonates, a smaller proportion of filtered sodium is absorbed in the proximal tubule and a correspondingly larger proportion delivered distally. The sodium retention that characterises growth is due to the influence of very high RAAS activity ( ). The preterm neonate has a reduced capacity to retain sodium owing to impaired reabsorption at the proximal tubule, resulting in a higher distal sodium delivery, and to limited aldosterone responsiveness at the distal tubule ( ). Intestinal absorption, though also limited ( ), is an important route for sodium conservation, with stool sodium loss generally less than 10% of intake.

The limited capacity to excrete a sodium load is not due to a low GFR, because filtration in even the most immature neonate greatly exceeds the amount that is ultimately retained, but because acute sodium loading results in only a blunted fall in RAAS activity and a limited natriuretic response ( ). Homeostasis is dependent on the regulation of tubular reabsorption, a process that in turn is dependent upon the stage of developmental maturation.
Tubular epithelial cells are bound by an apical membrane that faces the tubular lumen, and a basolateral membrane that lines the lateral and basal intercellular space and faces the peritubular capillaries. The lipid bilayer of the cell membrane is poorly permeable and solutes cross the membrane via transporters. These are specialised proteins inserted into the cell membrane. Their regulation is complex and involves hormones, intracellular signalling systems, protein phosphorylation, and endo- and exocytosis, each of which undergoes developmental regulation. Na + /K + -ATPase is the enzyme responsible for active sodium transport in all eukaryotic cells. There are many forms of Na + /K + -ATPase, each encoded by specific groups of Na + /K + -ATPase genes. In renal tubular cells, Na + /K + -ATPase, present on the basolateral membrane, creates an electrochemical gradient which is the energy source for the co-transport, involving specific transporter proteins, of Na + and glucose, and Na + and amino acids, and the countertransport of Na + and H + across the luminal membrane ( ). The long-term regulation of sodium balance is brought about by changes in the abundance of sodium transporters. During ontogenesis, there are tissue-specific patterns of increase in activity accompanied by an increase in Na + /K + -ATPase mRNA ( ). Antenatal glucocorticoid treatment increases the abundance of Na + /K + -ATPase in both lungs and kidney and enhances the maturation of renal tubular transport.
The postnatal enhancement in sodium conservation is brought about by the increasing responsiveness of the distal tubule to aldosterone ( ; ) and by an increase in the abundance of Na + /K + -ATPase and transporter proteins ( ; ). The ability to excrete a sodium load also matures during development. An increase or decrease in activity of renal Na + /K + -ATPase is the final common pathway for the short-term regulation of natriuresis ( ). Downregulatory factors that cause natriuresis include atrial natriuretic peptide (ANP), dopamine and diuretics. Noradrenaline is an upregulatory factor, which results in sodium retention. The peptide regulatory factors bind to cell membrane receptors and exert their effects via a cascade of intracellular messengers. Developmental maturation of these intracellular signalling systems fine-tunes the regulation of sodium balance ( ; ; ; ; ). Clinical observations offer some substantiation of these in vitro studies. ANP stimulates membrane-bound guanylate cyclase, which leads to an increase in the intracellular second messenger, cyclic guanosine monophosphate (cGMP), generated from endogenous guanosine triphosphate. cGMP interacts with specific protein kinases which in turn catalyse the phosphorylation of several protein substrates and this finally leads to a biological effect such as inhibition of sodium reabsorption. In a study of preterm babies ( ) the ratio of urinary cGMP to ANP was found to increase exponentially in the first 3 days after birth and then to reach a plateau. The ratio of sodium excretion to cGMP continued to increase over the 10 days of the study. This suggests a postnatal maturation in the ANP/cGMP/sodium excretion cascade and thus an increasing postnatal ability to excrete sodium.
Potassium
Potassium is the principal intracellular cation. Total body potassium is approximately 46 mmol/kg, a value that is similar in babies and adults. Unrecognised negative potassium balance may be relatively common in neonates receiving intensive care as it is easy to mask a falling serum potassium concentration if blood samples are slightly haemolysed. Preterm neonates should receive a potassium intake of 2 mmol/kg/day, commencing within 48 hours of birth if urine output is satisfactory and there are no concerns about renal function. Most gastrointestinal disorders are also associated with sodium loss and the resulting increase in circulating aldosterone exacerbates potassium loss. Hypokalaemia is usually accompanied by a metabolic alkalosis. Causes of potassium depletion are listed in Table 35.1 .
Inadequate intake |
Gastrointestinal loss |
|
Renal loss |
With metabolic alkalosis |
|
Diuresis during recovery from acute renal failure |
The serum potassium may be spuriously elevated if there has been difficulty in obtaining the blood sample or a delay in processing it, as potassium is released from damaged cells. True hyperkalaemia occurs in the context of extensive tissue damage, shock and ischaemia, and in renal failure. The management of hyperkalaemia is discussed below.
Renal water handling: urine flow rate
As nutrition can be provided to babies only in liquid form, a high fluid intake is necessary and a high urine flow rate must be sustained to maintain water balance. This is achieved by a much greater fractional excretion of glomerular filtrate (Fe H2O ). In newborn babies, lower hydrostatic and osmotic forces across the peritubular space result in decreased proximal tubular reabsorption of filtered water and a greater proportion of water is delivered distally in comparison with in older subjects. Water reabsorption in the distal nephron is regulated by the antidiuretic hormone (ADH), arginine vasopressin (AVP). The ADH-dependent increase in water permeability is brought about by the insertion of water channels, the aquaporins (AQP), from an intracellular vesicular reservoir into the apical membranes of cells of the collecting ducts ( ), allowing the movement of water across the tubular membrane in response to the high concentration of the medullary interstitium. Not all AQP isoforms are expressed in the human kidney and there are differences in expression during development, but fetal animals and preterm babies are sensitive to ADH ( ). The regulation of collecting-duct water permeability by vasopressin is mediated by AQP2 expression.
The capacity to concentrate urine develops progressively during postnatal life. The adult kidney can concentrate urine to 1200–1400 mOsmol/kg water. Infants are normally in a state of marked anabolism and have a lower urea production rate than adults, hence maximum osmolality is of the order of 600–800 mOsmol/kg. This difference is due to a shorter loop of Henle, reduced tonicity of the medullary interstitium as urea concentrations are low because of the highly anabolic state of the rapidly growing infant, and reduced expression of AQP2 . A higher urine concentration, however, can be produced under conditions of severe dehydration stress, in response to antenatal glucocorticoid therapy ( ) and if the urea production rate is increased by high protein feeding. Very immature babies in the first days after birth may have more limited concentrating ability and hence become dehydrated while continuing to pass urine of low osmolality.
The capacity of the healthy term and preterm neonate to dilute urine matches that of adults (minimum urine osmolality 50 mOsmol/kg H 2 O) ( ; ), hence diluting ability is unlikely to limit water excretion. showed that healthy preterm babies are able to adjust water excretion appropriately from the second day after birth, when their daily intakes were varied between 95 and 200 ml/kg, with sodium intake remaining constant. The Fe H2O increased from a mean of 7.4% to 13.1% of the filtered volume with the higher intake. A similarly high Fe H2O in adults would result in a daily urine volume of over 20 litres. Of note is that there was no concomitant increase in the loss of sodium in the urine, an observation that refutes the widely held view that babies are unable to sustain a high urine flow without an inevitable increase in the loss of sodium.
Given a diluting and concentrating capacity that extends from 50 to 600 mOsmol/kg and a daily renal solute load of approximately 10–15 mOsmol/kg ( ), the maximum and minimum urine flow rates that can be achieved are 300 ml/kg/24 h (12 ml/kg/h) ( ) and 25 ml/kg/24 h (1 ml/kg/h) respectively. The latter value, which represents the minimum urine flow rate beyond which solute retention would result, is the justification for the use of this figure as a clinical indication of renal failure. Healthy infants, demand-fed on their mother’s milk, usually produce urine approximately isotonic to plasma at a rate of about 3 ml/kg/h. A urine osmolality that lies between 200 and 400 mOsmol/kg usually suggests that fluid intake is satisfactory.
Specific gravity is often measured in place of osmolality as it can easily be performed on the ward. The presence of glucose or protein (both often found in samples from sick preterm babies) will, however, falsely elevate the specific gravity. In addition, the relationship between specific gravity, as measured with a refractometer, and osmolality differs between the newborn and older children, so that, in the newborn, an osmolality of 400 mOsmol/kg is indicative of a specific gravity anywhere between 1020 and 1030 ( Fig. 35.4 ) ( ). Reagent strip test measurement of urinary specific gravity is unsatisfactory ( ).

As neonates do not empty their bladders completely on voiding and as 7% fail to void during the first 24 hours after birth, external urine collections of short duration may be inaccurate. Urine can be collected using adhesive urine bags, taking care to protect the skin. Collection into preweighed nappies or cotton wool balls is widely practised, but can be misleading, as evaporation, leading to volume loss and increased osmolality, may be appreciable. Catheterisation is practicable even in the tiniest of babies.
Other urinary indices
Dipstick urinalysis may be used as a screening test for proteinuria, haematuria and glycosuria. Bilirubin causes dark-yellow to brown discoloration and suggests a conjugated hyperbilirubinaemia. Dark brown or red urine usually suggests haematuria, but may be caused by bile pigments, haemoglobin, rifampicin, porphyrins or urates. Haematuria may occur in renal venous and arterial thrombosis, cortical and tubular necrosis, neoplasia, obstructive uropathy, coagulopathy, nephritis and infection. Haemoglobinuria results from intravascular haemolysis and should raise suspicion of transfusion of incompatible blood, T-antigen activation or clostridial infection. Urine microscopy is necessary to detect red blood cells, leukocytes and casts. Red blood cell casts imply renal parenchymal pathology. A clean catch, catheter or suprapubic aspirate of urine should contain fewer than 5 white blood cells per cm 3 ( ). Leukocyturia is most often caused by infection but pyrexia or any inflammatory process may also be responsible. Newborns normally excrete small amounts of protein ( ).
Acid–base balance
The normal pH range of extracellular fluid is 7.35–7.45, corresponding to an H + concentration of 35–45 mmol/l. The regulation of acid–base balance involves, in order of speed of response, body buffers, respiratory function and renal function. In the proximal tubular cells, carbon dioxide, derived from cellular metabolism or diffusion from the tubular lumen, combines with water to form carbonic acid. This dissociates to H + and HCO 3 − . The H + is actively pumped into the tubular lumen and combines with filtered bicarbonate to form carbonic acid, which dissociates to water and CO 2 . The CO 2 then diffuses back into the tubular cell to repeat the cycle. The net effect is that, for each hydrogen ion excreted, one bicarbonate ion is retained, so that bicarbonate reserves are continuously regenerated:
In mature subjects, bicarbonate is regenerated by this process to maintain a plasma concentration of about 25 mmol/l, but preterm babies have a lower threshold ( ). The capacity to excrete an acid load depends on the presence of urinary buffers. Hydrogen ions are excreted all along the nephron and combine with base buffers, chiefly phosphate, sulphate and ammonia, in the tubular fluid, when bicarbonate reabsorption is complete. In health, renal excretion is the only route for acid loss. Acid gain may arise from respiratory or metabolic disorders. Newborn infants can acidify urine to the same extent as healthy adults ( ).
Acidosis
Acidosis may be respiratory, metabolic or mixed. In respiratory failure, carbon dioxide retention shifts the equation above to the right, with an increase in carbonic acid. Renal compensation is accomplished over a period of several days, by an increase in hydrogen ion excretion and bicarbonate regeneration. Blood gas analysis will reveal a compensated respiratory acidosis, with a high P CO 2 , raised bicarbonate and a normal pH ( Fig. 35.5 ). In an otherwise normal subject, a fall in pH will stimulate hyperventilation, shift the carbonic acid equation to the left and increase CO 2 elimination. The features of a compensated metabolic acidosis are a low bicarbonate, low P CO 2 and normal pH. Infants with both respiratory disease and a metabolic acidosis will show a mixed picture, with a high P CO 2 , low bicarbonate and low pH. Renal tubular acidosis (RTA) is discussed below. In a ventilated infant acute respiratory acidosis is managed by increasing the tidal volume or respiratory rate to lower the P CO 2 . Once compensatory changes have taken place, lowering the P CO 2 will lead to a respiratory alkalosis, hence the importance of recognising if compensation has taken place and, in this case, targeting ventilation management upon achieving a normal pH, and not a normal P CO 2. Metabolic acidosis is due to an increase in acid or a decrease in base. The best measure of metabolic acidosis is the standard base excess (SBE) as this is independent of P CO 2 .

In cases of unexplained metabolic acidosis at any age, measure the anion gap (AG), the difference between the sodium concentration (sometimes summated with potassium) and the sum of the chloride and bicarbonate:
The normal value is 8–16 mmol/l or, if sodium and potassium concentrations are summated, about 4 mmol/l higher. The normal gap is due to unmeasured anions, mainly albumin, phosphate and small amounts of organic anions, including lactate. A raised anion gap is due to an increase in either one of the ‘normal’ unmeasured anions (e.g. lactate) or another organic anion normally present in no more than trace amounts (e.g. ketoacids, complex organic anions in various inborn errors of metabolism). In patients with a normal anion gap metabolic acidosis the fall in HCO 3 − is compensated for by an increase in Cl − .
A common cause of metabolic acidosis in neonatal intensive care is tissue hypoxia. Metabolic acidosis also occurs in renal failure, from amino acid intolerance during parenteral nutrition, and in inborn errors of metabolism. Hyperchloraemia may occur with some parenteral nutrition formulations. Chloride is predominantly an extracellular anion and the normal serum level is 90–110 mmol/l. Partly replacing chloride with acetate in parenteral nutrition reduces the risk of hyperchloraemic metabolic acidosis. Medications that affect proximal tubular bicarbonate regeneration include the carbonic anhydrase inhibitor acetazolamide.
Metabolic acidosis arising as a consequence of poor peripheral perfusion will respond to a saline bolus. Treatment with base, such as sodium bicarbonate or tris(hydroxymethyl)aminomethane, may be appropriate. The formula widely used to calculate the quantity to achieve complete correction is mmol/l base = 0.3 × bodyweight (kg) × SBE (mmol/l), with ‘half-correction’ obtained by dividing this by two. This assumes that the extracellular fluid volume (ECFV) is 20% of bodyweight and, as administered base equilibrates to some extent with the intracellular compartment, this factor is increased to 0.3. The inherent imprecision in this approach should be appreciated; ECFV is considerably higher in the neonate, and the volume of distribution and rate of acid accumulation cannot be estimated with any degree of certainty.
Alkalosis
Respiratory alkalosis results from hyperventilation and the P CO 2 will be low. The commonest cause in the neonatal unit is iatrogenic and occurs during assisted ventilation, particularly high-frequency oscillatory ventilation. This is dangerous and is further discussed in Chapter 27 part 2 .
Metabolic alkalosis is caused by gain of base, as in the injudicious use of sodium bicarbonate or from loss of acid. A common cause is loss of gastric acid and chloride in high intestinal obstruction, vomiting or failure to replace gastric aspirates. In the distal tubule and collecting duct, sodium is reabsorbed in exchange for either potassium or hydrogen ions, under the influence of aldosterone. If intracellular H + is low, potassium is preferentially lost and vice versa, explaining the association between alkalosis and hypokalaemia. Hypokalaemia is both a cause and a consequence of metabolic alkalosis. Chronic diuretic therapy results in metabolic alkalosis through multiple mechanisms. Causes of metabolic alkalosis are shown in Table 35.2 .
Gastric loss | Vomiting |
Pyloric stenosis and other high intestinal obstruction | |
Unreplaced gastric aspirates | |
Stool loss | Diarrhoea |
Bartter syndrome | Hypokalaemic metabolic alkalosis; may present with polyhydramnios, polyuria and dehydration |
Diuretic therapy | Secondary hyperaldosteronism due to volume depletion, chloride depletion, potassium depletion |
Hypokalaemia | |
Refeeding syndrome | Associated with restoration of homeostasis, intracellular water and electrolyte shift, and hypokalaemia |
Adrenal hypersecretion | Primary and secondary hyperaldosteronism |
Excessive bicarbonate administration |
Acute renal failure
Acute renal failure (ARF) is the syndrome that results from an abrupt reduction in GFR. The spectrum of renal injury extends from mild tubular dysfunction to acute tubular necrosis or cortical necrosis. There may be full recovery from acute renal failure, or injury may be irreversible and fatal, or partial, leading to renal impairment many years later.
Formerly most often seen in the context of severe respiratory disease, perinatal asphyxia, sepsis and necrotising enterocolitis have emerged as the most common predisposing causes in neonatal intensive care. Renal injury may arise as a result of reduced renal perfusion, ischaemic injury, myoglobinuria following rhabdomyolysis or haemoglobinuria due to intravascular haemolysis. Antenatal exposure to angiotensin-converting enzyme inhibitors and angiotensin receptor blockers may present as renal impairment in the newborn and should be suspected if there is accompanying undermineralisation of the calvarium ( ).
There is no agreed definition for ARF in the newborn, nor is this unreasonable as this is not a steady-state period. A practical approach is to consider the possibility of renal impairment if the expected postnatal decline in PCr does not occur ( Fig. 35.2 ) or if it remains persistently above 1.5 mg/dl (about 130 µmol/l) ( ).
Presentation, investigation and management
Anticipate problems. Careful monitoring of urine output should commence on admission to neonatal intensive care. Delayed recognition of oligoanuria will increase the risk of prerenal impairment progressing to intrinsic renal failure. In term asphyxiated babies restrict initial fluid intake to 30–40 ml/kg/day until the situation is clear. This will not result in dehydration as it is considerably more than their transepidermal insensible loss, which is around 12 ml/kg/day. Hypoglycaemia may be a problem at low infusion volumes, and hypertonic glucose, infused centrally, may be necessary. Intravenous sodium should be avoided.
Though non-oliguric ARF is probably more frequent than oliguric ARF and represents the mild end of the spectrum of renal injury ( ), it is infrequently diagnosed. Oligoanuria is the commonest presenting sign and may indicate impaired renal perfusion (prerenal failure), established renal failure with damage to the renal parenchyma or urinary obstruction. If the urine flow rate falls below 1 ml/kg/h, immediate investigation is required. Consider the clinical context and whether the problem is likely to be prerenal, renal or postrenal. Ultrasound examination of the renal tract will identify congenital abnormalities, obstructive lesions such as posterior urethral values, postoperative urinary retention and the large, swollen kidneys of renal venous thrombosis.
Radionuclide imaging studies are of little initial value in distinguishing between causes of ARF in the newborn. Clinical examination should include assessment of perfusion (capillary refill time, toe–core temperature gap, blood pressure, metabolic acidosis), presence of renal masses and bladder palpation. Renal underperfusion leads to the production of urine of ‘poor quality’ (high osmolality, high urea and creatinine and low sodium concentration) ( Table 35.3 ). Some authors suggest that the best indicator to distinguish prerenal from established renal failure in the oliguric neonate is the fractional excretion of sodium (FeNa). This is calculated from the sodium and creatinine concentrations of plasma (P) and a spot urine (U) sample:
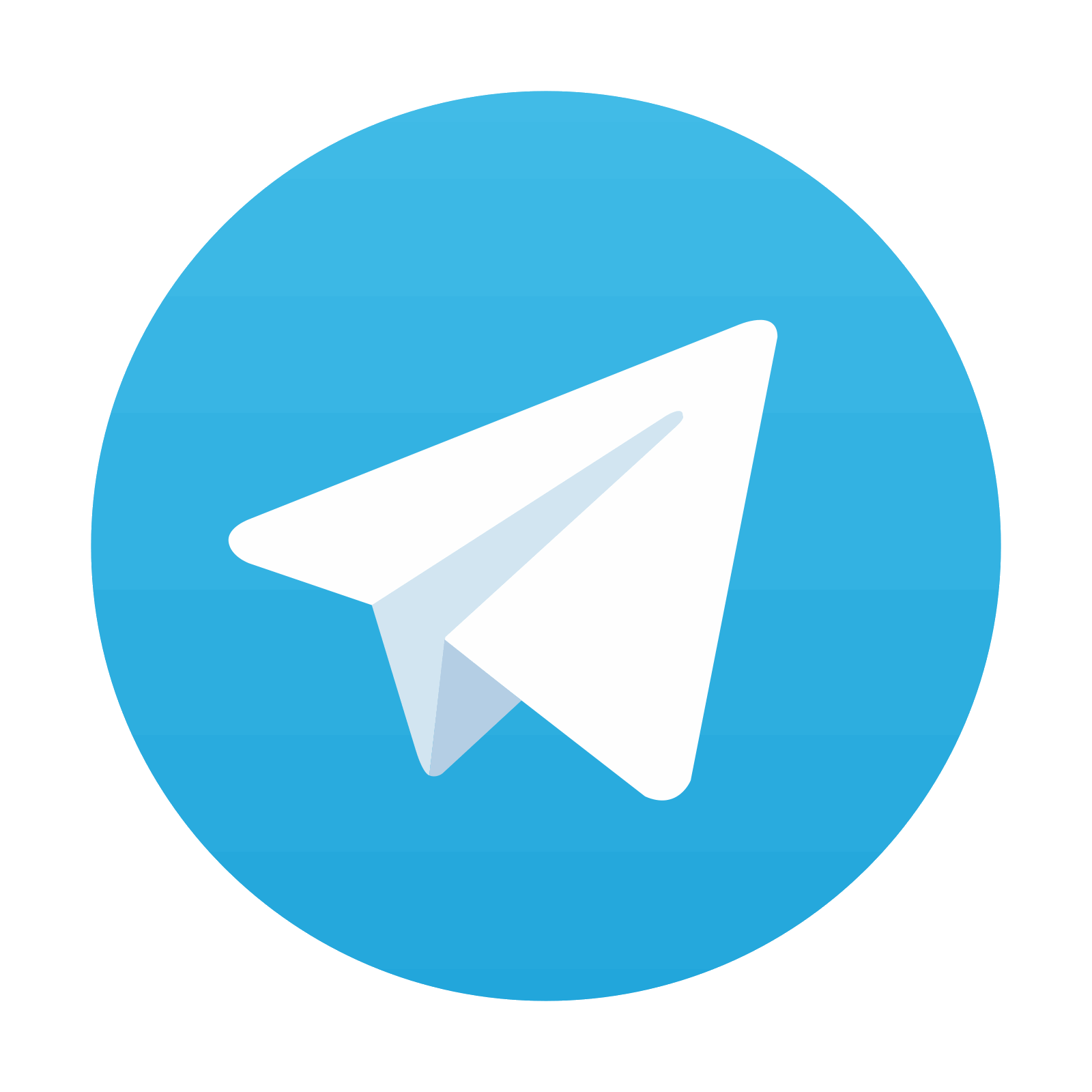
Stay updated, free articles. Join our Telegram channel
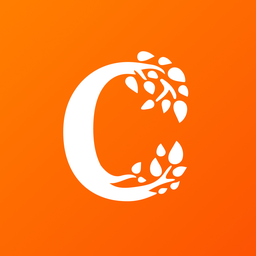
Full access? Get Clinical Tree
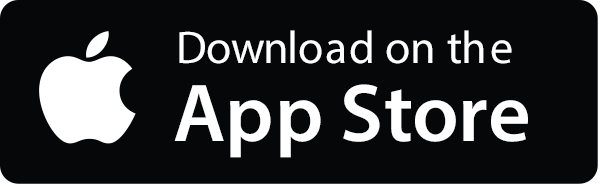
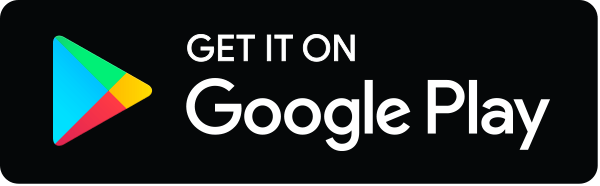