Chapter 583 Diabetes Mellitus
583.1 Introduction and Classification
Diabetes mellitus (DM) is a common, chronic, metabolic syndrome characterized by hyperglycemia as a cardinal biochemical feature. The major forms of diabetes are classified according to those caused by deficiency of insulin secretion due to pancreatic β-cell damage (type 1 DM, or T1DM) and those that are a consequence of insulin resistance occurring at the level of skeletal muscle, liver, and adipose tissue, with various degrees of β-cell impairment (type 2 DM, or T2DM). T1DM is the most common endocrine-metabolic disorder of childhood and adolescence, with important consequences for physical and emotional development. Individuals with T1DM confront serious lifestyle alterations that include an absolute daily requirement for exogenous insulin, the need to monitor their own glucose level, and the need to pay attention to dietary intake. Morbidity and mortality stem from acute metabolic derangements and from long-term complications (usually in adulthood) that affect small and large vessels resulting in retinopathy, nephropathy, neuropathy, ischemic heart disease, and arterial obstruction with gangrene of the extremities. The acute clinical manifestations are due to hypoinsulinemic hyperglycemic ketoacidosis. Autoimmune mechanisms are factors in the genesis of T1DM; the long-term complications are related to metabolic disturbances (hyperglycemia).
DM is not a single entity but rather a heterogeneous group of disorders in which there are distinct genetic patterns as well as other etiologic and pathophysiologic mechanisms that lead to impairment of glucose tolerance. A classification of diabetes and other categories of glucose intolerance is presented in Web Table 583-1. Three major forms of diabetes and several forms of carbohydrate intolerance are identified.
Web Table 583-1 ETIOLOGIC CLASSIFICATIONS OF DIABETES MELLITUS
* Patients with any form of diabetes may require insulin treatment at some stage of the disease. Such use of insulin does not, of itself, classify the patient.
Type 1 Diabetes Mellitus
Formerly called insulin-dependent diabetes mellitus (IDDM) or juvenile diabetes, T1DM is characterized by low or absent levels of endogenously produced insulin and dependence on exogenous insulin to prevent development of ketoacidosis, an acute life-threatening complication of T1DM. The natural history includes 4 distinct stages: (1) preclinical β-cell autoimmunity with progressive defect of insulin secretion, (2) onset of clinical diabetes, (3) transient remission “honeymoon period,” and (4) established diabetes associated with acute and chronic complications and decreased life expectancy. The onset occurs predominantly in childhood, with median age of 7-15 yr, but it may present at any age. The incidence of T1DM has steadily increased in many parts of the world, including Europe and the USA. T1DM is characterized by autoimmune destruction of pancreatic islet β cells. Both genetic susceptibility and environmental factors contribute to the pathogenesis. Susceptibility to T1DM is genetically controlled by alleles of the major histocompatibility complex (MHC) class II genes expressing human leukocyte antigens (HLAs). It is also associated with autoantibodies to islet cell cytoplasm (ICA), insulin (IAA), antibodies to glutamic acid decarboxylase (GADA or GAD65), and ICA512 (IA2). T1DM is associated with other autoimmune diseases such as thyroiditis, celiac disease, multiple sclerosis, and Addison disease. There is some suggestion that high dietary intake of omega-3 polyunsaturated fatty acids and vitamin D supplementation in early childhood decreases the incidence of autoimmune T1DM in at-risk children. In some children and adolescents with apparent T1DM, the β-cell destruction is not immune mediated. This subtype of diabetes occurs in patients of African or Asian origin and is distinct from known causes of β-cell destruction such as drugs or chemicals, viruses, mitochondrial gene defects, pancreatectomy, and ionizing radiation. These individuals may have ketoacidosis, but they have extensive periods of remission with variable insulin deficiency, similarly to patients with T2DM.
Type 2 Diabetes Mellitus
The children and adolescents with this type of diabetes are usually obese but are not insulin dependent and infrequently develop ketosis. Some may develop ketosis during severe infections or other stresses and may then need insulin for correction of symptomatic hyperglycemia. This category includes the most prevalent form of diabetes in adults, which is characterized by insulin resistance and often a progressive defect in insulin secretion. This type of diabetes was formerly known as adult-onset diabetes mellitus, non–insulin-dependent diabetes mellitus (NIDDM), or maturity-onset diabetes of the young (MODY).
The presentation of T2DM is typically more insidious than that with T1DM. In contrast to patients with T1DM who are usually ill at the time of diagnosis, children with T2DM often seek medical care because of excessive weight gain and fatigue as a result of insulin resistance and/or an incidental finding of glycosuria during routine physical examination. A history of polyuria and polydipsia is relatively uncommon in these patients. The incidence of T2DM in children has increased by more than 10-fold in many diabetes centers, in part as a result of the epidemic of childhood obesity (Chapter 44). Pediatric T2DM may account for as many as 30% of the new cases of diabetes, especially in obese African and Mexican-American adolescents. Acanthosis nigricans (dark pigmentation of skin creases/flexural areas), a sign of insulin resistance, is present in the majority of patients with T2DM and is accompanied by a relative hyperinsulinemia at the time of the diagnosis (Chapter 644). However, the serum insulin elevation is usually disproportionately lower than that of age-, weight-, and sex-matched nondiabetic children and adolescents, suggesting a state of insulin insufficiency. In some individuals, it may represent slowly evolving T1DM.
Other Specific Types of Secondary Diabetes
Examples include diabetes secondary to exocrine pancreatic diseases (cystic fibrosis), other endocrine diseases (Cushing syndrome), and ingestion of certain drugs or poisons (the rodenticide Vacor). Certain genetic syndromes, including those with abnormalities of the insulin receptor, also are included in this category. There are no associations with HLAs, autoimmunity, or islet cell antibodies among the entities in this subdivision.
Web Table 583-2 details the current criteria for the diagnosis of DM. It should be noted that a fasting blood glucose that exceeds 125 mg/dL (6.9 mmol/L) is the accepted criterion for the diagnosis of diabetes.
Web Table 583-2 DIAGNOSTIC CRITERIA FOR IMPAIRED GLUCOSE TOLERANCE AND DIABETES MELLITUS
IMPAIRED GLUCOSE TOLERANCE (IGT) | DIABETES MELLITUS (DM) |
---|---|
Fasting glucose 100-125 mg/dL (5.6-7.0 mmol/L) | Symptoms* of DM plus random plasma glucose ≥200 mg/dL (11.1 mmol/L) |
or | |
2-hr plasma glucose during the OGTT≥140 mg/dL, but <200 mg/dL 11.1 mmol/L) | Fasting plasma glucose ≥126 mg/dL (7.0 mmol/L)or2-hr plasma glucose during the OGTT ≥200 mg/dL |
OGTT, oral glucose tolerance test.
* Symptoms include polyuria, polydipsia, and unexplained weight loss with glucosuria and ketonuria.
From Report of the Expert Committee on the Diagnosis and Classification of Diabetes Mellitus, Diabetes Care 20(Suppl 1):S5, 1999.
Impaired Glucose Tolerance
The term impaired glucose tolerance (IGT) refers to a metabolic stage that is intermediate between normal glucose homeostasis and diabetes. A fasting glucose concentration of 99 mg/dL (5.5 mmol/L) is the upper limit of “normal.” This choice is near the level above which acute-phase insulin secretion is lost in response to intravenous administration of glucose and is associated with a progressively greater risk of the development of microvascular and macrovascular complications.
Many individuals with IGT (fasting glucose 100-125 mg/dL) are euglycemic in their daily lives and may have normal or nearly normal glycated hemoglobin levels. Individuals with IGT often manifest hyperglycemia only when challenged with the oral glucose load used in the standardized oral glucose tolerance test.
In the absence of pregnancy, IGT is not a clinical entity but rather a risk factor for future diabetes and cardiovascular disease. This may be observed as an intermediate stage in any of the disease processes listed in Web Table 583-1. IGT is often associated with the insulin resistance syndrome (also known as syndrome X or metabolic syndrome), which consists of insulin resistance, compensatory hyperinsulinemia to maintain glucose homeostasis, obesity (especially abdominal or visceral obesity), dyslipidemia of the high-triglyceride or low- or high-density lipoprotein type, or both, and hypertension (Chapter 44). Insulin resistance is directly involved in the pathogenesis of T2DM. IGT appears as a risk marker for this type of diabetes at least in part because of its correlation with insulin resistance. The diagnostic criteria for IGT are presented in Web Table 583-2.
583.2 Type 1 Diabetes Mellitus (Immune Mediated)
Epidemiology
T1DM accounts for about 10% of all diabetes, affecting 1.4 million in the USA and over 15 million in the world. While it accounts for most cases of diabetes in childhood, it is not limited to this age group; new cases continue to occur in adult life and approximately 50% of individuals with T1DM present as adults. The incidence of T1DM is highly variable among different ethnic groups. The overall age-adjusted incidence of type 1 DM varies from 0.7/100,000 per year in Karachi (Pakistan) to over 40/100,000 per year in Finland (Fig. 583-1). This represents a more than 400-fold variation in the incidence among 100 populations. The incidence of T1DM is increasing in most (but not all) populations and this increase appears to be most marked in populations where the incidence of autoimmune diseases was historically low. Data from Western European diabetes centers suggest that the annual rate of increase in T1DM incidence is 2-5%, whereas some central and eastern European countries demonstrate an even more rapid increase. The rate of increase is greatest among the youngest children. In the USA, the overall prevalence of diabetes among school-aged children is about 1.9/1,000, increasing from a prevalence of 1/1,430 children at 5 yr of age to 1/360 children at 16 yr. Among African Americans, the occurrence of T1DM is 30-60% of that seen in American whites. The annual incidence of new cases in the USA is about 14.9/100,000 of the child population. It is estimated that 30,000 new cases occur each year in the USA, affecting 1 in 300 children and as many as 1 in 100 adults during the lifespan. Rates are similar or higher in most Western European countries and significantly lower in Asia and Africa. But while incidence rates are much higher in European populations, the absolute number of new cases is almost equal in Asia and Europe because the population base is so much larger in Asia. Thus it is estimated that of the 400,000 total new cases of type 1 diabetes occurring annually in all children under age 14 yr in the world, about half are in Asia even though the incidence rates in that continent are much lower, because the total number of children in Asia is larger.
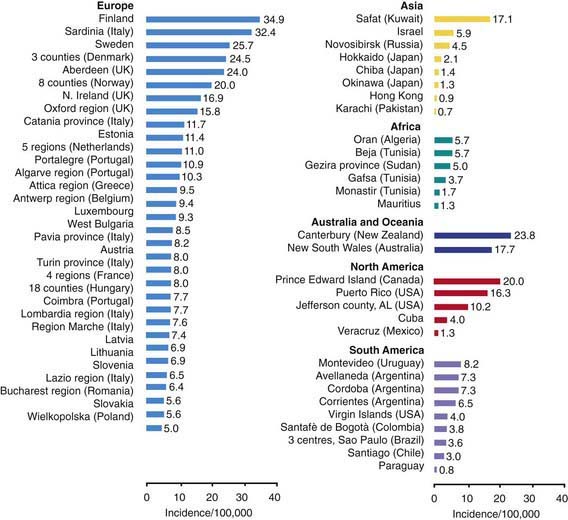
Figure 583-1 Incidence rates of type 1 diabetes mellitus by region and country.
(From Karvonen M, Viik-Kajander M, Moltchanova E, et al: Incidence of type I diabetes worldwide. Diabetes Mondiale (DiaMond) Project Group, Diabetes Care 23:1516–1526, 2000.)
Girls and boys are almost equally affected but there is a modest female preponderance in some low-risk populations (e.g., the Japanese); there is no apparent correlation with socioeconomic status. Peaks of presentation occur in 2 age groups: at 5-7 yr of age and at the time of puberty. The 1st peak may correspond to the time of increased exposure to infectious agents coincident with the beginning of school; the 2nd peak may correspond to the pubertal growth spurt induced by gonadal steroids and the increased pubertal growth hormone secretion (which antagonizes insulin). These possible cause-and-effect relationships remain to be proved. A growing number of cases are presenting between 1 and 2 yr of age, especially in high-risk groups; the average age of presentation is older in low-risk populations. Low-risk groups that migrate to a high-risk country seem to acquire an increased risk; for example, the children of Pakistani immigrants in the United Kingdom (UK) have an incidence rate similar to the local English population and 20 fold higher than the rates in Pakistan. On the other hand, there can be marked differences in incidence rates in various ethnic groups within the same country; for example, incidence rates in the 10-14 yr age group in the USA range from a low of 7.1 in Native Americans, to 17.6 in Hispanics, 19.2 in African-Americans, and 32.9 in whites. These variations also remain unexplained at this time.
Genetics
There is a clear familial clustering of T1DM, with prevalence in siblings approaching 6% while the prevalence in the general population in the USA is only 0.4%. Risk of diabetes is also increased when a parent has diabetes and this risk differs between the 2 parents; the risk is 2% if the mother has diabetes, but 7% when the father has diabetes. In monozygotic twins, the concordance rate ranges from 30-65%, whereas dizygotic twins have a concordance rate of 6-10%. Since the concordance rate of dizygotic twins is higher than the sibling risk, factors other than the shared genotypes (for example the shared intrauterine environment) may play a role in increasing the risk in dizygotic twins. Furthermore, the genetic susceptibility for T1DM in the parents of a child with diabetes is estimated at 3%. It should be kept in mind that although there is a large genetic component in T1DM, 85% of newly diagnosed type 1 diabetic patients do not have a family member with T1DM. Thus, we cannot rely on family history to identify patients who may be at risk for the future development of T1DM as most cases will develop in individuals with no such family history.
Monogenic Type 1 Diabetes Mellitus
Classic single gene defects are an extremely rare cause of type 1 diabetes, but they are not unknown. In 2 rare syndromes (IPEX and APS-1) the genetic susceptibility that leads to diabetes is due to a classic single gene defect. The IPEX (immune dysfunction, polyendocrinopathy, enteropathy, X-linked) syndrome is caused by mutations of the FOXP3 gene. The FOXP3 (forkhead box P3) is a gene involved in immune system responses. A member of the FOX protein family, FOXP3 appears to function as the master regulator in the development and function of regulatory T cells. These mutations lead to the lack of a major population of regulatory T lymphocytes with resulting overwhelming autoimmunity and development of diabetes (as early as 2 days of age) in approximately 80% of the children with this disorder.
APS-I (autoimmune polyendocrinopathy syndrome type 1) is caused by mutations of the AIRE (autoimmune regulator) gene, leading to abnormalities in expression of peripheral antigens within the thymus and/or abnormalities of negative selection in the thymus. This results in widespread autoimmunity. Approximately 18% of children with this syndrome develop type 1a diabetes.
Genes Altering the Risk of Autoimmune Type 1 Diabetes Mellitus
Most patients with T1DM do not have single gene defects. Instead, their risk of developing T1DM is modified by the influence of several risk loci. The genomic region with by far the greatest contribution to the risk of T1DM is the major histocompatibility complex on chromosome 6. One other region that consistently shows up in genetic studies is the promoter region 5′ of the insulin gene on chromosome 11. Other studies have identified several other risk loci. These loci include insulin (INS), protein tyrosine phosphatase nonreceptor type 22 (PTPN22), protein tyrosine phosphatase nonreceptor type 2 (PTPN2), interleukin (IL)-2 receptor (CD25), a lectin-like gene (KIAA0350), v-erb-b2 erythroblastic leukemia viral oncogene homolog 3e (ERBB3e), cytotoxic T-lymphocyte antigen 4 (CTLA4), and interferon-induced with helicase C domain 1 (IFIH1). However, except for PTPN22, their contribution is relatively small, thus making them less useful for predicting the genetic risk of T1DM in a given individual.
MHC/HLA Encoded Susceptibility to Type 1 Diabetes Mellitus
The MHC is a large genomic region that contains a number of genes related to immune system function in humans. These genes are further divided into HLA class I, II, III, and IV genes. Class II genes are the ones most strongly associated with risk of T1DM, but as genetic studies become more detailed it is becoming apparent that some of the risk associated with various HLA types is due to variation in genes in HLA classes other than class II. Overall, genetic variation in the HLA region can explain 40-50% of the genetic risk of T1DM (Fig 583-2).
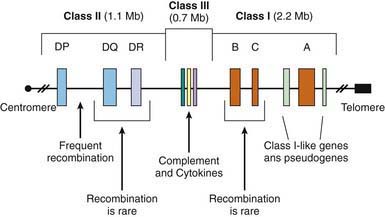
Figure 583-2 The human leukocyte antigen (HLA) complex (6p21.31). Graphic representation of the HLA complex, showing the relative locations the 3 classes of HLA genes.
(Courtesy of Dr. George Eisenbarth.)
Initially, much of the risk associated with diabetes appeared to be linked to DR3 and DR4 alleles, but the genes of the HLA locus display strong linkage disequilibrium and it is now known that some of the earlier identified risk alleles (like DR3/DR4) confer much of their increased risk because of their linkage with other alleles in the DQ region with which they are tightly linked with relatively low recombination rates.
Some of the known associations include the HLA DR3/4-DQ2/8 genotype; compared to a population prevalence of T1DM of approximately 1/300, DR3/4-DQ2/8 newborns from the general population have a 1/20 genetic risk. This risk of development of T1DM is even higher when the high-risk HLA haplotypes are shared with a sibling or parent with T1DM. Thus, if 1 sibling has T1DM and shares the same high-risk DR3/4-DQ2/8 haplotype with another sibling, then the risk of autoimmunity in the other sibling is 50%. And this risk approaches 80% when siblings share both HLA haplotypes identical by descent. This is known as the relative paradox and points to the existence of other shared genetic risk factors (most likely in the extended HLA haplotype).
With advances in genotyping, further discrimination is now possible and we can identify more specific risk ratios for specific haplotypes. For example, the DRB1*0401-DQA1*0301g-DQB1*0302 haplotype has an odds ratio (OR) of 8.39 while the DRB1*0401-DQA1*0301g-DQB1*0301 has an OR of 0.35, implicating the DQB1*0302 allele as a critical susceptibility allele. There are some dramatically protective DR-DQ haplotypes (e.g., DRB1*1501-DQA1*0102-DQB1*0602 [OR = 0.03], DRB1*1401-DQA1*0101-DQB1*0503 [OR = 0.02], and DRB1*0701-DQA1*0201-DQB1*0303 [OR = 0.02]). The DR2 haplotype (DRB1*1501-DQA1*0102-DQB1*0602) is dominantly protective and is present in 20% of general population but is seen in only 1% of type 1A diabetes patients.
Role of Aspartate at Position 57 in DQB1
DQB1*0302 (high risk for diabetes) differs from DQB1*0301 (protective against diabetes) only at position 57, where it lacks an aspartic acid residue. The DQB1*0201 allele (increased risk for diabetes) also lacks aspartic acid at position 57, and it has been proposed that the presence of aspartate at this position alters the protein recognition and protein binding characteristics of this molecule. But while the absence of aspartate at this position appears to be important in most studies on white individuals, it does not have the same role in Korean and Japanese populations. Moreover, certain low-risk DQB1 genotypes also lack aspartic acid at position 57, including DQB1*0302/DQB1*0201 (DR7), and DQB1*0201 (DR3)/DQB1*0201 (DR7). Thus, the presence of aspartate at this position is usually, but not always, protective in white populations, but not necessarily in other populations.
Role of HLA Class I
While the alleles of class II HLA genes appear to have the strongest associations with diabetes, recent genotyping studies and analyses of pooled data have identified associations with other elements in the HLA complex, especially HLA-A and HLA-B. The most significant association is with HLA-B39, which confers high risk for type 1A diabetes in 3 different populations, makes up the majority of the signal from HLA-B, and is associated with a lower age of onset of the disease.
Insulin Gene Locus, IDDM2
The 2nd locus found to be associated with risk of T1DM was labeled IDDM2 and has been localized to a region upstream of the insulin gene (5′ of the insulin gene). It is estimated that this locus accounts for about 10% of the familial risk of T1DM. Susceptibility in this region has been primarily mapped to a variable number of tandem repeats (VNTR) about 500 bp upstream of the insulin gene. This highly polymorphic region consists of anywhere from 30 to several hundred repeats of a 14-15 bp unit sequence (ACAGGGGTCTGGGG). Shorter number of repeats is associated with increased risk of T1DM.
PTPN22 (Lymphoid Tyrosine Phosphatase)
A single-nucleotide polymorphism (SNP) in the PTPN22 gene on chromosome 1p13 that encodes lymphoid tyrosine phosphatase (Lyp) correlates strongly with the incidence of T1DM in 2 independent populations. Since then, this discovery had been replicated in several populations and the gene has been found to have association with several other autoimmune diseases.
CTLA-4
The cytotoxic T lymphocyte–antigen 4 (CTLA-4) gene is located on chromosome 2q33 and has been found to be associated with type 1 diabetes risk as well as the risk of other autoimmune disorders in several studies. This gene is a negative regulator of T-cell activation and therefore is a good biologic candidate for type 1 diabetes risk modification.
Interleukin-2 (IL-2) Receptor
SNPs in or near the gene for the IL-2 receptor have been found to have an association with T1DM risk.
Interleukin-1 (IL-1) Receptor
IL-1 receptor activation and chemokines involved in monocyte/macrophage and neutrophil chemotaxis, have been also identified as critical steps in nitric oxide (NO)-induced islet necrosis and subsequent apoptosis. Indeed, inhibition of the activation of IL-1β–dependent inflammatory pathways by an IL-1 receptor antagonist in cultured rat islets exposed to NO prevented necrosis and apoptosis supporting evaluation in human islets in vitro and potentially as a post-transplant therapy.
Interferon-Induced Helicase
Another gene identified as having a modest effect on the risk of type 1 diabetes is the interferon-induced helicase (IFIH1) gene. This gene is thought to play a role in protecting the host from viral infections and given the specificity of different helicases for different RNA viruses, it is possible that knowledge of this gene locus will help to narrow down the list of viral pathogens that may have a role in type 1 diabetes.
CYP27B1
Cytochrome p450, subfamily 27, polypeptide 1 gene encodes vitamin D 1α-hydroxylase. Because of the known role of vitamin D in immune regulation and because of epidemiologic evidence that vitamin D may play a role in T1DM, this gene was examined as a candidate gene and 2 SNPs were found to be associated.
Other Genes
Several other genes (e.g., protein tyrosine phosphatase nonreceptor type 2 [PTPN-2]) and linkage blocks, including 2 linkage blocks on chromosome 12 (12q13 and 12q24) and blocks on 16p13, 18p11 and 18q22 have been found to be significant in genome-wide association studies and further fine mapping and functional studies of genes in these regions are pending.
Environmental Factors
The fact that 50% or so of monozygotic twins are discordant for T1DM, the variation seen in urban and rural areas populated by the same ethnic group, the change in incidence that occurs with migration, the increase in incidence that has been seen in almost all populations in the last few decades, and the occurrence of seasonality all provide evidence that environmental factors also play a significant role in the causation of T1DM.
Viral Infections
It is possible that various viruses do play a role in the pathogenesis of T1DM, but no single virus, and no single pathogenic mechanism, stands out in the environmental etiology of T1DM. Instead, a variety of viruses and mechanisms may contribute to the development of diabetes in genetically susceptible hosts.
Congenital Rubella Syndrome
The clearest evidence of a role for viral infection in human T1DM is seen in congenital rubella syndrome (CRS). Prenatal infection with rubella is associated with β-cell autoimmunity in up to 70%, with development of T1DM in up to 40% of infected children. The time lag between infection and development of diabetes may be as high as 20 yr. Type 1 diabetes after congenital rubella is more likely in patients that carry the higher risk genotypes. Interestingly, there appears to be no increase in risk of diabetes when rubella infection develops after birth, or when live-virus rubella immunization is used. Exactly how rubella infection leads to diabetes and why it is pathogenic only if infection occurs prenatally, remains unknown.
Enteroviruses
Studies have shown an increase in evidence of enteroviral infection in patients with T1DM and an increased prevalence of enteroviral RNA in prenatal blood samples from children who subsequently developed T1DM. In addition, there are case reports of association between enteroviral infection and subsequent T1DM. But the true significance of these infections remains unknown at this time.
Mumps Virus
It has been observed that mumps infection leads to the development of β-cell autoimmunity with high frequency and to T1DM in some cases. It has also been noted that there is an uptick in the incidence of T1DM 2-4 yr after an epidemic of mumps infection. But a large European study did not find any association between mumps infection and subsequent development of diabetes. Mumps vaccination, on the other hand, appears to be protective against diabetes. But while mumps may play a role in some cases of diabetes, the fact that T1DM diabetes incidence has increased steadily in several countries after universal mumps vaccination was introduced, and that incidence is extremely low in several populations where mumps is still prevalent, indicates that mumps is not an important causal factor in diabetes.
Role of Childhood Immunizations
Several large-scale well-designed studies have conclusively shown that routine childhood immunizations do NOT increase the risk of T1DM. On the contrary, immunization against mumps and pertussis has been shown to decrease the risk of T1DM.
The Hygiene Hypothesis: Possible Protective Role of Infections
While some viral infections may increase the risk of T1DM, infectious agents may also play a protective role against diabetes. The hygiene hypothesis states that lack of exposure to childhood infections may somehow increase an individual’s chances of developing autoimmune diseases, including T1DM. Epidemiologic patterns suggest that this may indeed be the case. Rates of T1DM and other autoimmune disorders are generally lower in underdeveloped nations with high prevalence of childhood infections, and tend to increase as these countries become more developed. The incidence of T1DM differs almost 6-fold between Russian Karelia and Finland even though both are populated by a genetically related population and are located next to each other at the same latitude. The incidence of autoimmunity in the 2 populations varies inversely with IgE antibody levels, and IgE is involved in the response to parasitic infestation. All these observations indicate that decreased exposure to certain parasites and other microbes in early childhood may lead to an increased risk of autoimmunity in later life, including autoimmune diabetes. On the other hand, retrospective case-control studies have been equivocal at best and direct evidence of protection by childhood infections is still lacking.
Diet
Breast-feeding may lower the risk of T1DM, either directly or by delaying exposure to cow’s milk protein. Early introduction of cow’s milk protein and early exposure to gluten have both been implicated in the development of autoimmunity and it has been suggested that this is due to the “leakiness” of the immature gut to protein antigens. Antigens that have been implicated include β-lactoglobulin, a major lipocalin protein in bovine milk, which is homologous to the human protein glycodelin (PP14), a T-cell modulator. Other studies have focused on bovine serum albumin as the inciting antigen, but the data are contradictory and not yet conclusive.
Other dietary factors that have been suggested at various times as playing a role in diabetes risk include omega-3 fatty acids, vitamin D, ascorbic acid, zinc, and vitamin E. Vitamin D is biologically plausible (it has a role in immune regulation), deficiency is more common in northern countries like Finland, and there is some epidemiologic evidence that decreased vitamin D levels in pregnancy or early childhood may be associated with diabetes risk; but the evidence is not yet conclusive and it is hoped that ongoing studies like TEDDY (The Environmental Determinants of Diabetes in the Young) will help to resolve some of the uncertainties in this area.
Psychologic Stress
Several studies show an increased prevalence of stressful psychologic situations among children who subsequently developed T1DM. Whether these stresses only aggravate pre-existing autoimmunity or whether they can actually trigger autoimmunity, remains unknown.
Role of Insulin Resistance: The Accelerator Hypothesis
The accelerator hypothesis proposes that T1DM and T2DM are the same disorder of insulin resistance, set against different genetic backgrounds. This “strong statement” of the accelerator hypothesis has been criticized as ignoring the abundant genetic and clinical evidence that the 2 diseases are distinct. Still, the hypothesis has focused attention on the role of insulin resistance and obesity in T1DM and there is evidence that the incidence of T1DM is indeed higher in children who exhibit more rapid weight gain. Whether this is simply another factor that stresses the β cell in the course of a primarily autoimmune disorder, or whether T1DM and T2DM can really be regarded as the same disease, is still open to question.
Pathogenesis and Natural History of Type 1 Diabetes Mellitus
In type 1A diabetes mellitus, a genetically susceptible host develops autoimmunity against his or her own β cells. What triggers this autoimmune response remains unclear at this time. In some (but not all) patients, this autoimmune process results in progressive destruction of β cells until a critical mass of β cells is lost and insulin deficiency develops. Insulin deficiency in turn leads to the onset of clinical signs and symptoms of T1DM. At the time of diagnosis, some viable β cells are still present and these may produce enough insulin to lead to a partial remission of the disease (honeymoon period) but over time, almost all β cells are destroyed and the patient becomes totally dependent on exogenous insulin for survival (Fig. 583-3). Over time, some of these patients develop secondary complications of diabetes that appear to be related to how well-controlled the diabetes has been. Thus, the natural history of T1DM involves some or all of the following stages:
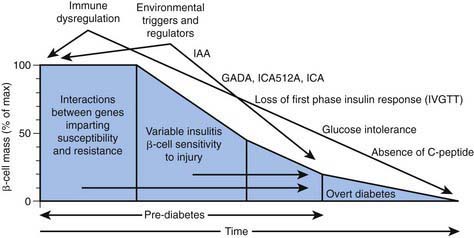
Figure 583-3 Proposed model of the pathogenesis and natural history of type 1 diabetes mellitus. IAA, insulin autoantibodies; GADA, glutamic acid decarboxylase antibody; ICA, islet cell antibody; IVGTT, intravenous glucose tolerance test.
(Adapted from Atkinson MA, Eisenbarth GS: Type 1 diabetes: new perspectives on disease pathogenesis and treatment, Lancet 358:221–229, 2001.)
Initiation of Autoimmunity
Genetic susceptibility to T1DM is determined by several genes (see section on genetics), with the largest contribution coming from variants in the HLA system. But it is important to keep in mind that even with the highest risk haplotypes, most carriers will NOT develop T1DM. Even in monozygotic twins, the concordance is 30-65%. A number of factors including prenatal influences, diet in infancy, viral infections, lack of exposure to certain infections, even psychologic stress, have been implicated in the pathogenesis of T1DM, but their exact role and the mechanism by which they trigger or aggravate autoimmunity remains uncertain (Fig. 583-4). What is clear is that markers of autoimmunity are much more prevalent than clinical T1DM, indicating that initiation of autoimmunity is a necessary but not a sufficient condition for T1DM. Whatever the triggering factor, it seems that in most cases of T1DM that are diagnosed in childhood, the onset of autoimmunity occurs very early in life. In a majority of the children diagnosed before age 10 yr, the 1st signs of autoimmunity appear before age 2 yr. Development of autoimmunity is associated with the appearance of several autoantibodies. Insulin associated antibodies (IAA) are usually the 1st to appear in young children, followed by glutamic acid decarboxylase 65 kd (GAD65) and tyrosine phosphatase insulinoma-associated 2 (IA-2) antibodies. The earliest antibodies are predominantly of the IgG1 subclass. Not only is there “spreading” of autoimmunity to more antigens (IAA, and then GAD 65 and IA-2) but there is also epitope spreading within 1 antigen. Initial GAD65 antibodies tend to be against the middle region or the carboxyl-terminal region, while amino-terminal antibodies usually appear later and are less common in children.
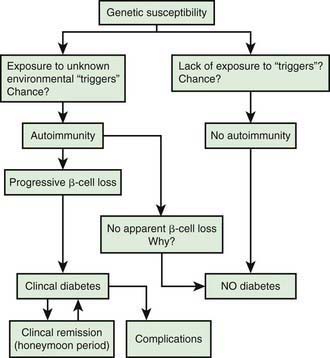
Figure 583-4 Schematic representation of the natural history of type 1 diabetes mellitus. Unknown triggers act upon a genetically susceptible host to trigger autoimmunity. Some proportion of those with autoimmunity develop progressive β-Cell loss that eventually leads to clinical diabetes. This is followed by temporary clinical remission (honeymoon period) in most patients. Over time, insulin secretion is almost completely lost and complications may develop in some patients (in direct proportion to the occurrence of hyperglycemia).
Preclinical Autoimmunity with Progressive Loss of β-Cell Function
In some, but not all patients, the appearance of autoimmunity is followed by progressive destruction of β cells. Antibodies are a marker for the presence of autoimmunity, but the actual damage to the β cells is primarily T-cell mediated (Fig. 583-5). Histologic analysis of the pancreas from patients with recent-onset T1DM reveals insulitis, with an infiltration of the islets of Langerhans by mononuclear cells, including T and B lymphocytes, monocytes/macrophages, and natural killer (NK) cells. In the NOD mouse, a similar cellular infiltrate is followed by linear loss of β cells until they completely disappear. But it appears that the process in human T1DM is not necessarily linear and there may be an undulating downhill course in the development of T1DM.
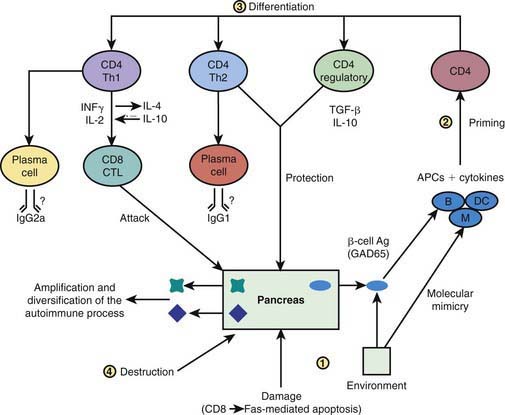
Figure 583-5 Schematic representation of the autoimmune response against pancreatic β cells. An insult to the pancreas leads to the release of β-cell antigens (GAD65), which are taken up by antigen-presenting cells (APCs) and the epitopes presented to the CD4 T cells. Type and stages of activation of APCs as well as the cytokine environment, in which the CD4 T cell priming takes place, dictate the differentiation of autoreactive T cells toward diabetogenic T helper-1 (Th1) cells, Th2 cells, or antigen-specific regulatory T cells. A predominant Th1 autoimmune response results in the recruitment and differentiation of cytotoxic CD8 cells, which attack the pancreatic β cells, leading to a massive release of β-cell antigens (Ag), epitope spreading, and destruction of the pancreatic islets. B, B lymphocyte; DC, dendritic cell; M, macrophage; CTL, cytotoxic cell; TGF-β, tumor growth factor-β; INFγ, interferon-γ; IL, interleukin.
(Adapted from Casares S, Brumeanu TD: Insights into the pathogenesis of T1DM: a hint for novel immunospecific therapies, Curr Mol Med 1:357–378, 2001.)
Role of Autoantibodies
The risk of developing clinical disease increases dramatically with an increase in the number of antibodies; only 30% of children with 1 antibody will progress to diabetes, but this risk increases to 70% when 2 antibodies are present and 90% when 3 are present. The risk of progression also varies with the intensity of the antibody response and those with higher antibody titers are more likely to progress to clinical disease. Another factor that appears to influence progression of β-cell damage is the age at which autoimmunity develops; children in whom IAAs appeared within the 1st 2 yr of life rapidly developed anti–islet cell antibodies and progressed to diabetes more frequently than children in whom the 1st antibodies appeared between ages 5 and 8 yr.
Role of Genetics in Disease Progression
Genetics plays a role in progression to clinical disease. In a large study of healthy children, the appearance of single antibodies is relatively common and usually transient, and does not correlate with the presence of high-risk HLA alleles, but those carrying high-risk HLA alleles are more likely to develop multiple antibodies and progress to disease. Similarly, the appearance of antibodies is more likely to predict diabetes in those with a family history of diabetes versus those with no family history of T1DM. Thus, it may be the case that environmental factors can induce transient autoimmunity in many children, but those with genetic susceptibility are more likely to see progression of autoimmunity and eventual development of diabetes.
Role of Environmental Factors
In addition to genetic factors, environmental factors may also act as accelerators of T1DM after the initial appearance of autoimmunity. This is evident from the fact that the incidence of T1DM can vary severalfold between populations that have the same prevalence of autoimmunity. For instance, the incidence of T1DM in Finland is almost 4-fold higher than in Lithuania, but the incidence of autoimmunity is similar in both countries.
The fact that all children with evidence of autoimmunity do not progress to diabetes indicates that there are “checkpoints” at which the autoimmune process can be halted or reversed before it progresses to full-blown diabetes. This has raised the possibility of preventing T1DM by intervening in the preclinical stage.
Onset of Clinical Disease
Patients with progressive β-cell destruction will eventually present with clinical T1DM. It was thought that 90% of the total β-cell mass is destroyed by the time clinical disease develops, but later studies have revealed that this is not always the case. It now appears that β-cell destruction is more rapid and more complete in younger children, while in older children and adults the proportion of surviving β cells is greater (10-20% in autopsy specimens) and some β cells (about 1% of the normal mass) survive up to 30 yr after the onset of diabetes. Since autopsies are usually done on patients who died of diabetic ketoacidosis, these figures may underestimate the actual β-cell mass present at diagnosis. Functional studies indicate that up to 40% of the insulin secretory capacity may be preserved in adults at the time of presentation of T1DM. The fact that newly diagnosed diabetic individuals may still have significant surviving β-cell mass is important because it raises the possibility of secondary prevention of T1DM. Similarly, the existence of viable β cells years or decades after initial presentation indicates that even longstanding diabetic patients may be able to exhibit some recovery of β-cell function if the autoimmune destructive process can be halted.
Prediction and Prevention
Autoimmunity precedes clinical T1DM, and indicators of maturing autoimmune responses may be useful markers for disease prediction. Individuals at risk for T1DM can be identified by a combination of genetic, immunologic, and metabolic markers. The most informative genetic locus, HLA class II, confers about half of the total genetic risk but has a low positive predictive value (PPV) when used in the general population. Autoantibodies provide a practical readout of β-cell autoimmunity, are easily sampled in venous blood, and have become the mainstay of T1DM prediction efforts. In the first-degree relatives of patients with T1DM, the number of positive d-aab can help estimate the risk of developing T1DM: low risk (single d-aab: PPV of 2-6%), moderate risk (2 d-aab: PPV of 21-40%), and high risk (>2 d-aab: PPV of 59-80%) over a 5 yr period. In children carrying the T1DM highest-risk genotype (HLA-DQB1*0201-DQA1*05/DQB1*0302-DQA1*03), insulitis is almost 10 times more frequent (PPV 21%) than in children with other genotypes (PPV 2.2%). But while autoantibodies are useful for detecting developing T1DM in close relatives of diabetic patients, most cases are sporadic rather than familial, necessitating general population screening. This has been difficult, in part, because the observed autoantibody prevalence greatly exceeds the low disease prevalence in nonrelatives, leading to high false-positive rates.
Primary Prevention of Type 1 Diabetes Mellitus
A safe, effective, inexpensive, and easily administered intervention could theoretically be targeted at all newborns, but no such universally effective intervention is yet available. Delaying the introduction of cow’s milk protein, delaying introduction of cereals, and increasing the duration of breast-feeding are all potentially beneficial and trials of these interventions are ongoing. But the fact that the disease has continued to increase in incidence in Northern Europe while breast-feeding has increased indicates that these interventions may not be sufficient to reverse the epidemic. Other dietary interventions that are being tested, or may be tested in high-risk subjects, include supplementing omega-3 fatty acids and vitamin D, and taking cod liver oil during pregnancy. In all these cases, there are some hints of possible benefit but nothing has been conclusively proven at this point.
In high-risk populations (relatives of individuals with T1DM, especially those with high-risk genotypes), it is feasible to test more targeted interventions. One of the 1st interventions to be tested in a high-risk population was the use of nicotinamide supplementation, but this failed to prevent T1DM. Parenteral insulin and nasal insulin proved similarly ineffective in preventing diabetes, but oral insulin appeared to delay the incidence of diabetes in some patients. A larger trial of oral insulin is currently ongoing and results are awaited. Other studies that are ongoing or planned will look at the effect of GAD-alum and anti-CD3 antibodies in subjects at high risk for the development of T1DM. Results of these trials are awaited.
Secondary Prevention
Type 1 diabetes is a T-cell-mediated autoimmune disease that begins, in many cases, 3-5 yr before the onset of clinical symptoms, continues after diagnosis, and can recur after islet transplantation. The effector mechanisms responsible for the destruction of β cells involve cytotoxic T cells as well as soluble T-cell products, such as interferon-γ and tumor necrosis factor-α. Such observations have led to clinical trials with immunomodulatory drugs. Depending on age, anywhere from 10-20% to 40% (or more) of a person’s β cells may be intact at the time of diagnosis. In addition, small numbers of β cells may survive (or develop anew) up to 30 yr after diagnosis. This raises the possibility that diabetes can be cured or ameliorated by stopping the autoimmune destructive process after initial diagnosis (secondary prevention).
Immunosuppressants like cyclosporine have been tested for this purpose, but while they may prolong the honeymoon period, they are associated with significant side effects and are only effective as long as they are being administered, so their use for this purpose has been abandoned. Trials using CD3 antibodies have been more promising, but some patients developed flulike symptoms and reactivation of Epstein-Barr virus infection. Further trials of this therapy and other therapies targeted at various components of T cells and β cells are planned or ongoing.
The possibility of using glucagon-like peptide (GLP-1) agonists (e.g., exenatide) alone or in combination with immunomodulatory therapies is also being explored as these agents are capable of increasing β cell mass in animals.
Pathophysiology
Insulin performs a critical role in the storage and retrieval of cellular fuel. Its secretion in response to feeding is exquisitely modulated by the interplay of neural, hormonal, and substrate-related mechanisms to permit controlled disposition of ingested foodstuff as energy for immediate or future use. Insulin levels must be lowered to then mobilize stored energy during the fasted state. Thus, in normal metabolism, there are regular swings between the postprandial, high-insulin anabolic state and the fasted, low-insulin catabolic state that affect liver, muscle, and adipose tissue (Table 583-1). T1DM is a progressive low-insulin catabolic state in which feeding does not reverse but rather exaggerates these catabolic processes. With moderate insulinopenia, glucose utilization by muscle and fat decreases and postprandial hyperglycemia appears. At even lower insulin levels, the liver produces excessive glucose via glycogenolysis and gluconeogenesis, and fasting hyperglycemia begins. Hyperglycemia produces an osmotic diuresis (glycosuria) when the renal threshold is exceeded (180 mg/dL; 10 mmol/L). The resulting loss of calories and electrolytes, as well as the persistent dehydration, produce a physiologic stress with hypersecretion of stress hormones (epinephrine, cortisol, growth hormone, and glucagon). These hormones, in turn, contribute to the metabolic decompensation by further impairing insulin secretion (epinephrine), by antagonizing its action (epinephrine, cortisol, growth hormone), and by promoting glycogenolysis, gluconeogenesis, lipolysis, and ketogenesis (glucagon, epinephrine, growth hormone, and cortisol) while decreasing glucose utilization and glucose clearance (epinephrine, growth hormone, cortisol).
TABLE 583-1 INFLUENCE OF FEEDING (HIGH INSULIN) OR OF FASTING (LOW INSULIN) ON SOME METABOLIC PROCESSES IN LIVER, MUSCLE, AND ADIPOSE TISSUE*
HIGH PLASMA INSULIN (POSTPRANDIAL STATE) | LOW PLASMA INSULIN (FASTED STATE) | |
---|---|---|
Liver | Glucose uptake | Glucose production |
Glycogen synthesis | Glycogenolysis | |
Absence of gluconeogenesis | Gluconeogenesis | |
Lipogenesis | Absence of lipogenesis | |
Absence of ketogenesis | Ketogenesis | |
Muscle | Glucose uptake | Absence of glucose uptake |
Glucose oxidation | Fatty acid and ketone oxidation | |
Glycogen synthesis | Glycogenolysis | |
Protein synthesis | Proteolysis and amino acid release | |
Adipose tissue | Glucose uptake | Absence of glucose uptake |
Lipid synthesis | Lipolysis and fatty acid release | |
Triglyceride uptake | Absence of triglyceride uptake |
* Insulin is considered to be the major factor governing these metabolic processes. Diabetes mellitus may be viewed as a permanent low-insulin state that, untreated, results in exaggerated fasting.
The combination of insulin deficiency and elevated plasma values of the counter-regulatory hormones is also responsible for accelerated lipolysis and impaired lipid synthesis, with resulting increased plasma concentrations of total lipids, cholesterol, triglycerides, and free fatty acids. The hormonal interplay of insulin deficiency and glucagon excess shunts the free fatty acids into ketone body formation; the rate of formation of these ketone bodies, principally β-hydroxybutyrate and acetoacetate, exceeds the capacity for peripheral utilization and renal excretion. Accumulation of these keto acids results in metabolic acidosis (diabetic ketoacidosis, DKA) and compensatory rapid deep breathing in an attempt to excrete excess CO2 (Kussmaul respiration). Acetone, formed by nonenzymatic conversion of acetoacetate, is responsible for the characteristic fruity odor of the breath. Ketones are excreted in the urine in association with cations and thus further increase losses of water and electrolyte. With progressive dehydration, acidosis, hyperosmolality, and diminished cerebral oxygen utilization, consciousness becomes impaired, and the patient ultimately becomes comatose.
Clinical Manifestations
As diabetes develops, symptoms steadily increase, reflecting the decreasing β-cell mass, worsening insulinopenia, progressive hyperglycemia, and eventual ketoacidosis. Initially, when only insulin reserve is limited, occasional hyperglycemia occurs. When the serum glucose increases above the renal threshold, intermittent polyuria or nocturia begins. With further β-cell loss, chronic hyperglycemia causes a more persistent diuresis, often with nocturnal enuresis, and polydipsia becomes more apparent. Female patients may develop monilial vaginitis due to the chronic glycosuria. Calories are lost in the urine (glycosuria), triggering a compensatory hyperphagia. If this hyperphagia does not keep pace with the glycosuria, loss of body fat ensues, with clinical weight loss and diminished subcutaneous fat stores. An average, healthy 10 yr old child consumes about 50% of 2,000 daily calories as carbohydrate. As that child becomes diabetic, daily losses of water and glucose may be 5 L and 250 g, respectively, representing 1,000 calories, or 50%, of the average daily caloric intake. Despite the child’s compensatory increased intake of food, the body starves because unused calories are lost in the urine.
When extremely low insulin levels are reached, keto acids accumulate. At this point, the child quickly deteriorates. Keto acids produce abdominal discomfort, nausea, and emesis, preventing oral replacement of urinary water losses. Dehydration accelerates, causing weakness or orthostasis—but polyuria persists. As in any hyperosmotic state, the degree of dehydration may be clinically underestimated because intravascular volume is conserved at the expense of intracellular volume. Ketoacidosis exacerbates prior symptoms and leads to Kussmaul respirations (deep, heavy, rapid breathing), fruity breath odor (acetone), prolonged corrected Q-T interval (QTc), diminished neurocognitive function, and possible coma. About 20-40% of children with new-onset diabetes progress to DKA before diagnosis.
This entire progression happens much more quickly (over a few weeks) in younger children, probably owing to more aggressive autoimmune destruction of β cells. In infants, most of the weight loss is acute water loss because they will not have had prolonged caloriuria at diagnosis, and there will be an increased incidence of DKA at diagnosis. In adolescents, the course is usually more prolonged (over months), and most of the weight loss represents fat loss due to prolonged starvation. Additional weight loss due to acute dehydration may occur just before diagnosis. In any child, the progression of symptoms may be accelerated by the stress of an intercurrent illness or trauma, when counter-regulatory (stress) hormones overwhelm the limited insulin secretory capacity.
Diagnosis
The diagnosis of T1DM is usually straightforward. Although most symptoms are nonspecific, the most important clue is an inappropriate polyuria in any child with dehydration, poor weight gain, or “the flu.” Hyperglycemia, glycosuria, and ketonuria can be determined quickly. Nonfasting blood glucose greater than 200 mg/dL (11.1 mmol/L) with typical symptoms is diagnostic with or without ketonuria. In the obese child, T2DM must be considered (see Type 2 Diabetes Mellitus, later). Once hyperglycemia is confirmed, it is prudent to determine whether DKA is present (especially if ketonuria is found) and to evaluate electrolyte abnormalities—even if signs of dehydration are minimal. A baseline hemoglobin A1C (HbA1c) allows an estimate of the duration of hyperglycemia and provides an initial value by which to compare the effectiveness of subsequent therapy.
In the nonobese child, testing for autoimmunity to β cells is not necessary. Other autoimmunities associated with T1DM should be sought, including celiac disease (by tissue transglutaminase IgA and total IgA) and thyroiditis (by antithyroid peroxidase and antithyroglobulin antibodies). Because significant physiologic distress can disrupt the pituitary-thyroid axis, free thyroxine (T4) and thyroid-stimulating hormone (TSH) levels should be checked after the child is stable for a few weeks.
Rarely, a child has transient hyperglycemia with glycosuria while under substantial physical stress. This usually resolves permanently during recovery from the stressors. Stress-produced hyperglycemia can reflect a limited insulin reserve temporarily revealed by counter-regulatory hormones. A child with temporary hyperglycemia should therefore be monitored for the development of symptoms of persistent hyperglycemia and tested if such symptoms occur. Formal testing in a child who remains clinically asymptomatic is not necessary.
Routine screening procedures, such as postprandial determinations of blood glucose or screening oral glucose tolerance tests, have yielded low detection rates in healthy, asymptomatic children, even among those considered at risk, such as siblings of diabetic children. Accordingly, such screening procedures are not recommended in children.
Diabetic Ketoacidosis
DKA is the end result of the metabolic abnormalities resulting from a severe deficiency of insulin or insulin effectiveness. The latter occurs during stress as counter-regulatory hormones block insulin action. DKA occurs in 20-40% of children with new-onset diabetes and in children with known diabetes who omit insulin doses or who do not successfully manage an intercurrent illness. DKA may be arbitrarily classified as mild, moderate, or severe (Table 583-2), and the range of symptoms depends on the depth of ketoacidosis. There is a large amount of ketonuria, an increased ion gap, a decreased serum bicarbonate (or total CO2) and pH, and an elevated effective serum osmolality, indicating hypertonic dehydration.
Treatment
Therapy is tailored to the degree of insulinopenia at presentation. Most children with new diabetes (60-80%) have mild to moderate symptoms, have minimal dehydration with no history of emesis, and have not progressed to ketoacidosis. Once DKA has resolved in the newly diagnosed child, therapy is transitioned to that described for children with nonketotic onset. Children with previously diagnosed diabetes who develop DKA are usually transitioned to their previous insulin regimen.
New-Onset Diabetes without Ketoacidosis
Excellent diabetes control involves many goals: to maintain a balance between tight glucose control and avoiding hypoglycemia, to eliminate polyuria and nocturia, to prevent ketoacidosis, and to permit normal growth and development with minimal effect on lifestyle. Therapy encompasses initiation and adjustment of insulin, extensive teaching of the child and caretakers, and reestablishment of the life routines. Each aspect should be addressed early in the overall care. Ideally, therapy can begin in the outpatient setting, with complete team staffing by a pediatric endocrinologist, experienced nursing staff, dietitians with training as diabetes educators, and a social worker. Close contact between the diabetes team and family must be assured. Otherwise, initial therapy should be done in the hospital setting.
Insulin Therapy
Several factors influence the initial daily insulin dose per kilogram of body weight. The dose is usually higher in pubertal children. It is higher in those who have to restore greater deficits of body glycogen, protein, and fat stores and who, therefore, have higher initial caloric capacity. On the other hand, most children with new-onset diabetes have some residual β-cell function (the honeymoon period), which reduces exogenous insulin needs. Children with long-standing diabetes and no insulin reserve require about 0.7 U/kg/day if prepubertal, 1.0 U/kg/day at midpuberty, and 1.2 U/kg/day by the end of puberty. A reasonable dose in the newly diagnosed child, then, is about 60-70% of the full replacement dose based on pubertal status. The optimal insulin dose can only be determined empirically, with frequent self-monitored blood glucose levels and insulin adjustment by the diabetes team. Residual β-cell function usually fades within a few months and is reflected as a steady increase in insulin requirements and wider glucose excursions.
The initial insulin schedule should be directed toward the optimal degree of glucose control in an attempt to duplicate the activity of the β cell. There are inherent limits to our ability to mimic the β cell. Exogenous insulin does not have a 1st pass to the liver, whereas 50% of pancreatic portal insulin is taken up by the liver, a key organ for the disposal of glucose; absorption of an exogenous dose continues despite hypoglycemia, whereas endogenous insulin release ceases and serum levels quickly lower with a normally rapid clearance; and absorption rate from an injection varies by injection site and patient activity level, whereas endogenous insulin is secreted directly into the portal circulation. Despite these fundamental physiologic differences, acceptable glucose control can be obtained with new insulin analogs used in a basal-bolus regimen, that is, with slow-onset, long-duration background insulin for between-meal glucose control and rapid-onset insulin at each meal.
All preanalog insulins form hexamers, which must dissociate into monomers subcutaneously before being absorbed into the circulation. Thus, a detectable effect for regular (R) insulin is delayed by 30-60 min after injection. This, in turn, requires delaying the meal 30-60 min after the injection for optimal effect—a delay rarely attained in a busy child’s life. R has a wide peak and a long tail for bolus insulin (Figs. 583-6 and 583-7). This profile limits postprandial glucose control, produces prolonged peaks with excessive hypoglycemic effects between meals, and increases the risk of nighttime hypoglycemia. These unwanted between-meal effects often necessitate “feeding the insulin” with snacks and limiting the overall degree of blood glucose control. NPH and Lente insulins also have inherent limits because they do not create a peakless background insulin level (see Fig. 583-7C-E). This produces significant hypoglycemic effect during the midrange of their duration. Thus, it is often difficult to predict their interaction with fast-acting insulins. When R is combined with NPH or Lente (see Fig. 583-7E), the composite insulin profile poorly mimics normal endogenous insulin secretion. There are broad areas of excessive insulin effect alternating with insufficient effect throughout the day and night. Lente and Ultralente insulins have been discontinued and are no longer available.
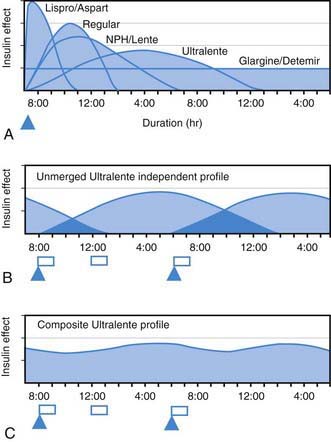
Figure 583-6 Approximate insulin effect profiles. Meals are shown as rectangles below time axis. A, The following relative peak effect and duration units are used: lispro/aspart, peak 20 for 4 hr; regular, peak 15 for 7 hr; NPH/Lente, peak 12 for 12 hr; Ultralente, peak 9 for 18 hr; glargine, peak 5 for 24 hr. Though Lente and Ultralente are no longer manufactured, they are shown to give historical comparison to newer insulin analogs. , Injection time. B, Two Ultralente injections given at breakfast and supper. Note overlap of profiles. C, Composite curve showing approximate cumulative insulin effect for the 2 Ultralente injections. This composite view is much more useful to the patient, parents, and medical personnel because it shows important combined effects of multiple insulin injections with variable absorption characteristics and overlapping durations.
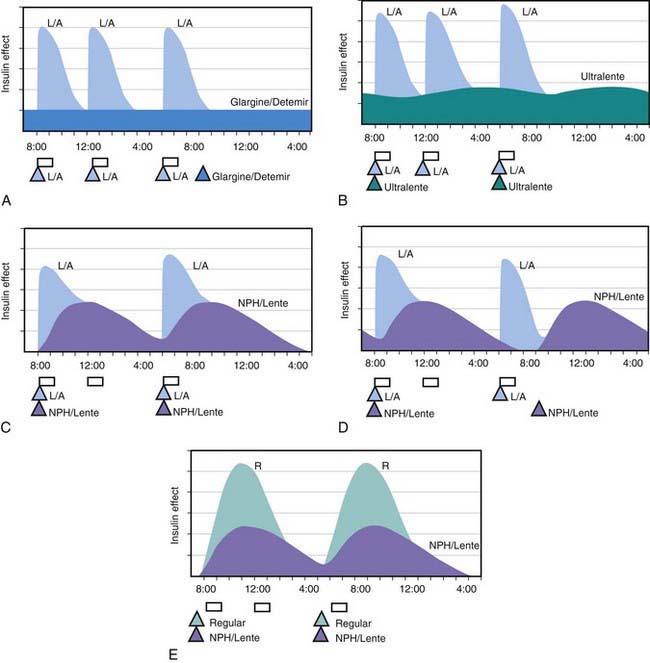
Figure 583-7 Approximate composite insulin effect profiles. Meals are shown as rectangles below time axis. Injections are shown as labeled triangles. L/A, lispro or aspart. Even though the fast- and long-acting insulins are shaded differently to show the addition of 1 insulin effect to another, the profile is changed to show the combined effect. For example, in the breakfast injection in C, the quick decline of L/A effect is blunted by the rising NPH/Lente effect, producing a broad tail, which slowly declines to baseline at supper. All profiles are idealized using average absorption and clearance rates. In typical clinical situations, these profiles vary among patients. A given patient has varying rates of absorption depending on the injection site, physical activity, and other variables. A, L or A pre-meal; glargine at bedtime. The rapid onset and short duration of L or A reduce overlap between pre-meal injections, and there is no extended nighttime action. This reduces the risk of hypoglycemia. Glargine provides a steady basal profile that simplifies prediction of bolus insulin effect. B, L or A pre-meal; Ultralente at breakfast and supper. Ultralente produces a basal profile similar to that seen with glargine. Some excessive insulin effect, however, is seen before supper and at nighttime. C, L or A pre-meal; NPH or Lente at breakfast and supper. The broad peak of NPH or Lente produces substantial risk of hypoglycemia before lunch and during the early hours of the night. The waning insulin effect before supper and breakfast may also allow breakthrough hyperglycemia. D, L or A pre-meal; NPH or Lente at breakfast and bedtime. Moving the evening long-acting insulin helps to cover the pre-breakfast hours, but the risk of nighttime lows persists. E, Regular and NPH or Lente at breakfast and supper. This produces the least physiologic profile, with large excesses before lunch and during the early night, combined with poor coverage before supper and breakfast. Though Lente and Ultralente are no longer manufactured, they are shown to give historical comparison to newer insulin analogs.
Lispro (L) and aspart (A), insulin analogs, are absorbed much quicker because they do not form hexamers. They provide discrete pulses with little if any overlap and short tail effect. This allows better control of post-meal glucose increase and reduces between-meal or nighttime hypoglycemia (see Fig. 583-7A). The long-acting analog glargine (G) creates a much flatter 24-hr profile, making it easier to predict the combined effect of a rapid bolus (L or A) on top of the basal insulin, producing a more physiologic pattern of insulin effect (see Fig. 583-7A). Postprandial glucose elevations are better controlled, and between-meal and nighttime hypoglycemia are reduced.
Ultralente (UL) given twice a day provided a reasonable basal profile (see Fig. 593-6C) and was quite effective when used with lispro or aspart (see Fig. 583-7B). Since UL is no longer available, G may be given every 12 hr in young children if a single daily dose of G does not produce complete 24-hr basal coverage. The basal insulin glargine should be 25-30% of the total dose in toddlers and 40-50% in older children. The remaining portion of the total daily dose is divided evenly as bolus injections for the 3 meals. A simple 3- or 4-step dosing schedule is begun based on the blood glucose level (Table 583-3). As soon as the family is taught to calculate the carbohydrate content of meals, bolus insulin can be more accurately dosed by both the carbohydrate content of the meal as well as the ambient glucose (see Table 583-3).
Frequent blood glucose monitoring and insulin adjustment are necessary in the 1st weeks as the child returns to routine activities and adapts to a new nutritional schedule, and as the total daily insulin requirements are determined. The major physiologic limit to tight control is hypoglycemia. Intensive control dramatically reduces the risk of long-term vascular complications; it is associated with a 3-fold increase in severe hypoglycemia. Use of insulin analogs moderates but does not eliminate this problem.
Some families may be unable to administer 4 daily injections. In these cases, a compromise may be needed. A 3-injection regimen combining NPH with a rapid analog bolus at breakfast, a rapid-acting analog bolus at supper, and NPH at bedtime may provide fair glucose control. Further compromise to a 2-injection regimen (NPH and rapid analog at breakfast and supper) may occasionally be needed. However, such a schedule would provide poor coverage for lunch and early morning, and would increase the risk of hypoglycemia at midmorning and early night.
Insulin Pump Therapy
Continuous subcutaneous insulin infusion (CSII) via battery-powered pumps provides a closer approximation of normal plasma insulin profiles and increased flexibility regarding timing of meals and snacks compared with conventional insulin injection regimens. Insulin pump models can be programmed with a patient’s personal insulin dose algorithms, including the insulin to carbohydrate ratio and the correction scale for pre-meal glucose levels. The patient can enter his or her blood glucose level and the carbohydrate content of the meal, and the pump computer will calculate the proper insulin bolus dose. Insulin pump therapy in adolescents with T1DM is associated with improved metabolic control and reduced risk of severe hypoglycemia without affecting psychosocial outcomes. The use of overnight CSII improves the metabolic control in children aged 7-10 yr. CSII has also been useful in toddlers. CSII may not always improve metabolic control. Some studies show improvement in less than one half and worsening control (higher HbA1c) in one fifth of patients. It is likely that the degree of glycemic control is mainly dependent on how closely patients adhere to the principles of diabetes self-care, regardless of the type of intensive insulin regimen. One benefit of pump therapy may be a reduction in severe hypoglycemia and associated seizures. Randomized trials comparing multiple daily insulin (MDI) regimen using glargine insulin and CSII in children with T1DM demonstrate similar metabolic control and frequency of hypoglycemic events.
It is anticipated that existing subcutaneous glucose sensors and external insulin pumps can be linked with an insulin delivery algorithm to create a completely automated closed-loop system. The development of a closed-loop insulin pump technology is currently being evaluated and has been an active area of research over the past several years. The development of such a system, with particular emphasis on creating a system mimicking the physiologic properties of the β cell is highly desirable. Closed-loop glucose control using an external sensor and insulin pump provided a means to achieve near-normal glucose concentrations in youth with T1DM during the overnight period. The addition of small manual priming bolus doses of insulin, given 15 min before meals, improved postprandial glycemic excursions.
Inhaled and Oral Insulin Therapies
Preprandial inhaled insulin is being evaluated in adults with T1DM and T2DM. The preliminary metabolic data are promising. Patients taking pre-meal inhaled insulin in combination with once daily bedtime long-acting insulin (Ultralente) injection achieved similar metabolic control compared with patients taking 2-3 daily injections of insulin. There was no significant difference in the frequency of hypoglycemic episodes between the 2 groups. There have been reports of pulmonary fibrosis in a small number of patients, necessitating further monitoring and evaluation of patients taking inhaled insulin before this route of insulin administration is deemed safe. Bioavailability of inhaled insulin is increased with smoking and reduced with asthma.
Pre-meal oral insulin (Oralin) has been evaluated in comparison with oral hypoglycemic agents, mostly in patients with T2DM. The clinical data appear promising, but further evaluation of efficacy in T1DM is needed. In addition, pre-meal inhaled insulin (Exubera), a powder form of recombinant human insulin, has been evaluated for use in individuals with T1DM and T2DM. Although Exubera insulin was shown to be effective and safe in long-term clinical initial trials with minor risk of lung fibrosis and cancer in smokers, it was discontinued by Pfizer Pharmaceuticals in 2008 due to high cost to patients compared to subcutaneous insulin. Currently, other formulations of inhaled insulin are under investigations in clinical trials in patients with T1DM and T2DM.
Amylin-Based Adjunct Therapy
Pramlintide acetate, a synthetic analog of amylin, may be of therapeutic value combined with insulin therapy. In adolescents it has been shown to decrease postprandial hyperglycemia, insulin dosage, gastric emptying, and HbA1c levels. It is given as a subcutaneous dose before meals.
Basic and Advanced Diabetes Education
Therapy consists not only of initiation and adjustment of insulin dose but also of education of the patient and family. Teaching is most efficiently provided by experienced diabetes educators and nutritionists. In the acute phase, the family must learn the “basics,” which includes monitoring the child’s blood glucose and urine ketones, preparing and injecting the correct insulin dose subcutaneously at the proper time, recognizing and treating low blood glucose reactions, and having a basic meal plan. Most families are trying to adjust psychologically to the new diagnosis of diabetes in their child and thus have a limited ability to retain new information. Written materials covering these basic topics help the family during the 1st few days.
Children and their families are also required to complete advanced self-management classes in order to facilitate implementation of flexible insulin management. These educational classes will help patients and their families acquire skills for managing diabetes during athletic activities and sick days.
Ketoacidosis
Severe insulinopenia (or lack of effective insulin action) results in a physiologic cascade of events in 3 general pathways:
Reversal of DKA is associated with inherent risks that include hypoglycemia, hypokalemia, and cerebral edema. Any protocol must be used with caution and close monitoring of the patient. Adjustments based on sound medical judgment may be necessary for any given level of DKA (Table 583-4).
Table 583-4 DIABETIC KETOACIDOSIS (DKA) TREATMENT PROTOCOL
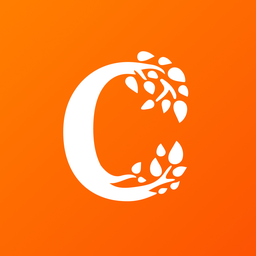
Full access? Get Clinical Tree
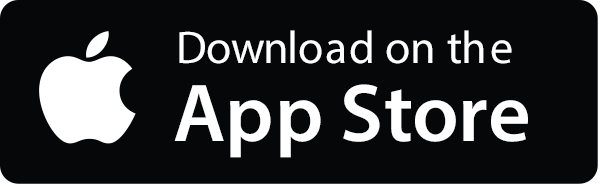
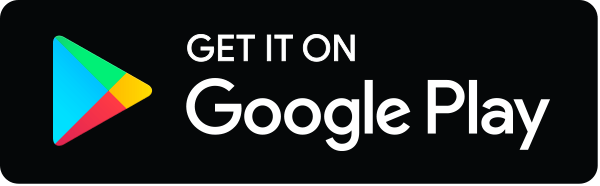