Developmental Physiology
The kidneys play a central role in the physiologic transition from fetal to postnatal life. Whereas homeostasis in utero is maintained largely by the placenta, adaptation to the extra uterine environment requires that the kidneys assume the responsibility of regulating water and solute balance. Although the neonatal kidney traditionally has been characterized as dysfunctional, closer analysis indicates that this organ functions at a level that is appropriate to the physiologic needs of the growing infant, except in the very-low-birth-weight (VLBW) infant.
Embryology
The metanephros, the definitive mammalian kidney, first appears at 5 weeks postconceptional age and begins to produce urine by 10 weeks postconceptional age (1,2 and 3). The ureteric bud, an offshoot of the mesonephric duct, induces formation of nephrons within the metanephric blastema. The nephroblastic cells of the blastema, on contact with the ureteric bud, differentiate into the glomerulus, proximal convoluted tubule, loop of Henle, and distal convoluted tubule. The ureteric bud ultimately forms the ureter, pelvis, calyces, and collecting ducts of the metanephros.
The processes of induction, morphogenesis and differentiation of the metanephros occurs in a centrifugal pattern, proceeding from the center to the periphery (4,5 and 6). Thus, the first nephrons to develop are those residing in the juxtamedullary region, whereas the youngest are located in the nephrogenic zone just under the renal capsule.
The full complement of approximately 1 million neph-rons per kidney in the human is achieved by about 35 weeks’ gestational age (GA), or a body weight of about 2,300 g (6). When birth occurs before this age, the formation of new nephrons (nephronogenesis) continues until a full complement is achieved. Once complete, nephronogenesis is never resumed, even after extensive loss of renal tissue. Thus, the full-term infant is born with as many nephrons as he or she will ever have.
Physiologic, biochemical, and enzymatic maturation of newly formed nephrons may lag behind anatomic maturation by weeks or months. Thus, the immature kidney is characterized by structural and functional heterogeneity arising from the concurrent presence of nephrons in varied stages of differentiation.
Renal Physiology
Urine produced by the fetal metanephric kidneys contributes to the formation of amniotic fluid (3,6). Although renal function is not necessary for long-term regulation of fetal water and electrolyte homeostasis, it is important for normal growth (7), lung development (8), and protection from acute changes in fetal vascular volume (9). The hourly rate of urine production in normal fetuses is about 5 mL at 20 weeks, 10 mL at 30 weeks, increasing to up to 50 mL at 40 weeks (3,10).
Renal Blood Flow
Low rates of fetal and neonatal renal blood flow (RBF) exist in a variety of animal species, whether normalized to body weight, surface area or kidney weight. RBF in the human fetus, estimated by Doppler ultrasonography, increases from 20 mL/min at 25 weeks GA to more than 60 mL/min at 40 weeks and reaches adult levels by 2 years of age (11,12). The effective renal plasma flow (RPF) has traditionally been calculated from the renal clearance of the organic acid para-aminohippurate (PAH), corrected for the fraction of PAH extracted by the kidney (12); the value for effective RPF divided by (100- hematocrit [%])/100 provides an estimate of RBF. The effective RPF increases rapidly between 30 and 40 weeks GA, reaching adult values by 24 months of postnatal life (12) (Fig. 42-1).
Because the renal extraction ratio for PAH varies with age, calculated values of effective RPF must be interpreted with caution in the fetus and neonate. The rate of extraction of PAH in the 1-week-old full-term newborn is only about 60%, reaching the adult value of 90% by 5 months (13). PAH clearance averages 148 mL/min/1.73 M2 body surface area (BSA) in full-term infants at 2 weeks of life, increasing to 200 mL/min/1.73 M2 by 3 months (14). PAH clearance, corrected for BSA, reaches adult levels sometime between 1 and 2 years of age (12). The incomplete PAH extraction early in life appears to be as a result of relatively greater blood flow to juxtamedullary nephrons and efferent arteriovenous shunting in cortical nephrons, thereby allowing blood to bypass PAH-transporting segments of the nephron. Additionally, the organic acid secretory pathways in the proximal tubule are immature early in development (15). Thus, RBF and RPF will be underestimated by PAH clearance alone in neonates, particularly in premature infants, in the absence of determination of the PAH extraction ratio.
![]() Figure 42-1 Developmental changes in effective renal plasma flow (ERPF), calculated from the renal clearance of para-aminohippurate (PAH) and corrected for the fraction of PAH extracted by the kidney. The ERPF, used to estimate RBF, increases rapidly between 30 and 40 weeks GA, reaching adult values by 24 months of postnatal life (From ref. 12, with permission). |
RBF is determined by cardiac output and the ratio of renal to systemic vascular resistance. Developmental changes in both hemodynamic variables contribute to the postnatal increase in RBF. Adult kidneys (which comprise 0.5% of total body mass) receive approximately 20% to 25% of the total cardiac output, corresponding to a RBF of 4 mL/min/g kidney weight. In contrast, the previable fetus receives only about 5% of the cardiac output, and the 1-week-old term infant receives about 9% (16).
The perinatal increase in RBF is gradual (13,17,18), consistent with the premise that clamping of the umbilical cord and the immediate imposition of functional demands at birth do not in themselves account for the postnatal increase in RBF. The maturational increase in RBF cannot be completely explained by increases in renal mass (19) or by nephronogenesis, which is complete well before maximal levels of RBF are achieved.
The intrarenal distribution of RBF in the newborn differs from that in the adult, reflecting the relative size, number, and maturity of glomeruli present in the different regions of the kidney at that stage of development. RBF in the newborn is distributed primarily to the inner cortex and medulla. Maturation is accompanied by a redistribution of blood flow toward the superficial cortex, so that the ratio of inner cortical to outer cortical blood flow becomes progressively smaller than in the fetus (18,20,21 and 22) (Fig. 42-2). At maturity, about 93% of RBF goes to the cortex, which constitutes about 75% of the renal mass, whereas only 7% is distributed to the renal medulla and perirenal fat.
![]() Figure 42-2 Postnatal changes in the intrarenal distribution of blood flow. Relative rates of blood flow per glomerulus in the four cortical zones of the canine kidney. Zone I represents the most superficial region, and zone IV represents the deepest. The total height of the bars in each age group is equal. At birth, the blood flow to the superficial cortex was lowest, with most blood flow perfusing the deep cortex. By 6 weeks of age, this pattern was reversed. Maturation is accompanied by an increase in blood flow to the outer cortex, due primarily to a decrease in renal vascular resistance (From ref. 17, with permission). |
The primary factor responsible for the maturational increase and change in intrarenal distribution in RBF is a decrease in renal vascular resistance (RVR) (23), with the rise in cardiac output and mean arterial blood pressure (MAP) only partially accounting for these postnatal changes in renal hemodynamics (23,24 and 25,27). The balance of afferent and efferent arteriolar resistances ultimately determines the RVR, RBF, glomerular capillary pressure and glomerular filtration rate (GFR). The intrarenal vascular resistance, localized both at the afferent and efferent arterioles (26), is much higher in the newborn than in the adult (19,23). The postnatal fall in RVR occurs at a time when the systemic vascular resistance increases about 6-fold (23).
Anatomic factors also contribute to the developmental increase and redistribution of RBF. The intrarenal vascular system distal to the afferent arteriole in the neonate differs from that in the adult. Variability in the complexity of the glomerular capillary network exists early in postnatal life (28). Inner cortical glomeruli at this age generally have a smaller number of capillaries than the in adult, although they appear similar in overall structure. Few efferent arterioles descend into the medulla; most connect directly to the venous system (arteriovenous shunting) (29), bypassing the proximal tubules (26).
A variety of vasoactive substances have been implicated in the developmental regulation of RBF and GFR. The most important factors are discussed below. Changes in the balance of the vasoconstrictor renin-angiotensin system (RAS) and renal sympathetic nervous systems, both of which are highly active in early development, and vasodilatory humoral factors, including nitric oxide (NO), are believed to mediate, at least in part, the developmental reduction in RVR and increase in RBF.
Renin-Angiotensin System
All components of the RAS are present in the fetal metanephric kidney (30,31,32,33,34) (Fig. 42-3). Renin, angiotensino- gen and angiotensin-converting enzyme (ACE) are first detected in fetal metanephros by ∼6 weeks gestation. The site of localization of renin within the kidney is developmental-stage specific. Whereas the majority of renin-containing cells are located in the juxtamedullary apparatus in both the newborn and adult, renin message and protein in the fetus are also present in the arcuate and interlobular arteries and glomeruli (35).
The RAS is very active in the fetus and newborn. The fetus produces renin as early as 17 weeks GA (36). Plasma renin activity (PRA) is inversely related to GA in the fetus and newborn, decreasing from 60 ng/mL/h at 30 weeks to about 10 to 20 ng/mL/h at term (37). PRA is 3-to-5 times higher in human infants (38,39,40,41,42,43 and 44) than in adults. Changes in PRA in the fetus in response to several stimuli (increase after volume depletion or hypoxia, and decrease after volume expansion or after administration of a β-adrenergic antagonist or of a prostaglandin synthetase inhibitor [PGSI]) are similar to those observed in adults.
The high levels of PRA in neonates are generally associated with circulating levels of angiotensin II (ANG II) and aldosterone that exceed those measured in the adult (45,46,47,48,49 and 50). These high levels may reflect high rates of secretion or low metabolic clearance rates relative to body size. The reason for these high levels may be a relative end-organ unresponsiveness to aldosterone (51,52). Circulating levels of plasma ANG II decrease during postnatal life in parallel with PRA (53).
The observation that administration of ACE inhibitors during late gestation can result in anuria and oligohydramnios, renal tubular abnormalities, pulmonary hypoplasia, growth retardation, and increased fetal loss (54,55,56) underscores the pivotal role of the RAS in normal fetal growth and development. Genetic disruption of the genes encoding angiotensinogen (57), ACE (58), or ANG II type 1 re-ceptor is also associated with profound abnormalities in kidney morphology.
SThe significance of the RAS in the maintenance of renal hemodynamics in the perinatal period remains uncertain. However, as identified above, a number of observations suggest that this axis may be important under conditions of stress in the near-term fetus. An acute reduction in blood volume or onset of hypoxia in the fetus or neonate significantly increases renin and ANG II levels (10,59,60). ACE inhibition blocks the increase in RVR and reduction in RBF experienced by near-term fetuses in response to hemorrhage (61,62).
Kinins
Bradykinins are potent vasodilator peptides generated from the protein precursor kininogen by the proteolytic enzyme kallikrein (Fig. 42-3). The kinins are inactivated by kininase I, which is a carboxypeptidase, and the peptidyl dipeptide hydrolase kininase II, which is ACE. Thus, ACE inhibitors not only decrease ANG II production but also prevent the breakdown of kinins.
Prostaglandins
Prostaglandins (PGs) contribute to the maintenance of RBF and GFR, especially during conditions of enhanced vasoconstrictor activity. Complex interactions between PGs and the RAS and kinin systems exist, making it difficult to identify the specific effects of PGs on regulation of blood pressure, RBF, and electrolyte and water homeostasis. PG synthesis from arachidonic acid requires cyclooxygenase (COX), the inhibitory target of aspirin and various non-steroidal antiinflammatory drugs (65). Two isoforms of COX have been identified, each representing a different gene product and subject to differential regulation. COX-1 has been proposed to participate in glomerulogenesis (66), whereas COX-2 regulates renal perfusion and glomerular hemodynamics (67). Differences in intrarenal COX-1 and -2 localization between the adult and fetal human kidney may account for the variable renal responses to PG inhibition observed between the two groups (66).
Urinary excretion of PGs is high in the fetus (68,69), presumably reflecting a high rate of renal synthesis. Urinary excretion of PGE2 and prostacyclin metabolites in the premature infant is five times that noted at term and 20 times that measured in older children (70). Although rates of excretion decrease after birth, urinary PG excretion remains significantly higher in neonates than in children or adults (71,72).
PGs produced by the fetal and neonatal kidney may contribute to the regulation of renal perfusion and glomerular hemodynamics. Maternal administration of indomethacin, a nonselective COX inhibitor, reduces RBF in the fetus, and is associated with an increase in RVR and MAP (73). Intrauterine exposure of the human fetus to COX inhibitors can lead to a decrease in GFR with consequent reduction in fetal urine output and oligohydramnios (74,75,76,77). Postnatal administration of a PGSI to preterm infants (78,79) to close a patent ductus arteriosus (PDA) may also compromise renal function, leading to a reduction in RBF, GFR and urine volume.
Renal Nerves and the Adrenergic System
The renal vascular bed of the fetus is less reactive to renal nerve stimulation than that of the newborn and adult (80,81). In contrast to observations made in adult animals, several studies have demonstrated that renal nerve stimulation in the fetal and newborn kidney activates noradrenergic pathways, norepinephrine stimulates β2-adrenoceptors to produce renal vasodilation, and maturation of the renal adrenergic system is associated with downregulation of the renal β-adrenoceptor response (80,81 and 82).
Circulating catecholamine levels, particularly norepinephrine, are very high just before and immediately after birth (83), falling to adult values within a few days of life. The high plasma levels of catecholamines act directly to increase afferent arteriolar tone and indirectly, via stimulation of renin and ANG II release, to increase efferent resistance, possibly contributing to the maintenance of the high RVR characteristic of the neonatal kidney (84). The fetal and neonatal kidney demonstrates enhanced sensitivity to catecholamines compared to the adult, related in part to developmental differences in adrenergic receptor density (85,86).
Nitric Oxide
Endogenous NO is a major vasodilator in the developing kidney, contributing to the maintenance of intrarenal vascular tone and serving to buffer the highly activated endogenous vasoconstrictor state (87,88). The sensitivity of renal hemodynamics in the fetal and neonatal kidney to inhibition of NO synthesis is significantly greater than that of the adult kidney.
Dopamine and Dopamine Receptors
The fetal and neonatal kidneys are less sensitive than their adult counterparts to the renal vasodilatory and the natriuretic effects of dopamine (89,90). Exogenous administration of dopamine, which results in an increase in RBF in the adult, has no effect in the young animal unless there also is an increase in systemic blood pressure and cardiac output (81,91,92). This blunted response is considered to reflect the limited generation of the vasodilatory second messenger cyclic adenosine monophosphate (cAMP) (89,91) and a low density of renal dopamine-1-like receptors in the neonatal kidney (93).
The pharmacological effects of dopamine are dose-dependent. Whereas the renal response to low concentrations of dopamine generally reflects dopaminergic receptor-mediated effects, high concentrations stimulate α-adrenergic receptors. Thus, the renal vasoconstriction observed following intrarenal dopamine infusion in fetal and neonatal animals (94,95) likely reflects dopamine binding to α-adrenoceptors, which are abundant by term (96,97).
Arginine Vasopressin
The plasma concentration of arginine vasopressin (AVP) in neonates increases abruptly after birth and is highest in infants whose mothers labored before vaginal delivery (98). Although AVP has the potential to contribute to the high RVR characteristic of the neonate through its action on vascular and glomerular V1 receptors, its role in regulating basal renal hemodynamics remains poorly defined. Infusion of synthetic AVP does not alter RBF and RVR in fetal sheep (99). However, AVP may play a role in certain stress-induced responses. For example, during hemorrhage, but not during hypoxemia, the marked decrease in RBF and increase in RVR correlate closely with the rise in plasma AVP (10,59,100).
Atrial Natriuretic Factor
ANF acts on the mature kidney to increase sodium excretion and GFR, antagonize renal vasoconstriction, and inhibit renin secretion (101). ANF release from atrial cardiocytes is stimulated in the fetus by an increase in intra-cardiac pressure and atrial distention (102,103,104 and 105), and levels fall in response to a decrease in central venous pressure, as during hemorrhage (106). The natriuretic and diuretic response to systemically infused ANF in newborns is attenuated compared to adults (107,108,109,110 and 111). Whereas specific ANF receptors have been identified on near-term fetal glomerular membranes, the ANF binding capacity is age-dependent, increasing 7-fold between fetal and adult life (112). The blunted response of the immature subject to ANF has been attributed to ineffective production of the second messenger cyclic GMP (108,113), a rapid systemic clearance of ANF (107,108), and a greater ANF-induced reduction in RBF in fetuses and newborns than in adults (107).
Glomerular Filtration
Initiation of glomerular filtration in the human fetus occurs between 9 and 12 weeks of GA (114,115). It has been suggested that the functional demand placed on the neonatal kidney by cessation of placental function at birth stimulates GFR to increase. However, estimates of GFR correlate well with GA, a relationship that persists whether the fetus remains in utero or is born prematurely (116,117,118 and 119). Specifically, GFR averages approximately 8 to 10 mL/min/ 1.73 M2 at 28 weeks of GA and increases only slightly before 34 weeks postmenstrual age, although body size and kidney weight increase appreciably during this time. After about 34 weeks postmenstrual age, at which time GFR averages 25 mL/min/1.73 M2 regardless of postnatal age, GFR increases rapidly, often by threefold to fivefold within 1 week (116,118,120), coincident with completion of nephronogenesis (Fig. 42-4). Thus, an infant born prematurely at 28 weeks of GA shows little increase in GFR until the infant is about 6 weeks old, i.e., until a postmenstrual age of 34 weeks is attained and nephrogenesis has been completed (121). The GFR of neonates who are small for GA is similar to that of infants whose body weight is appropriate for the same GA.
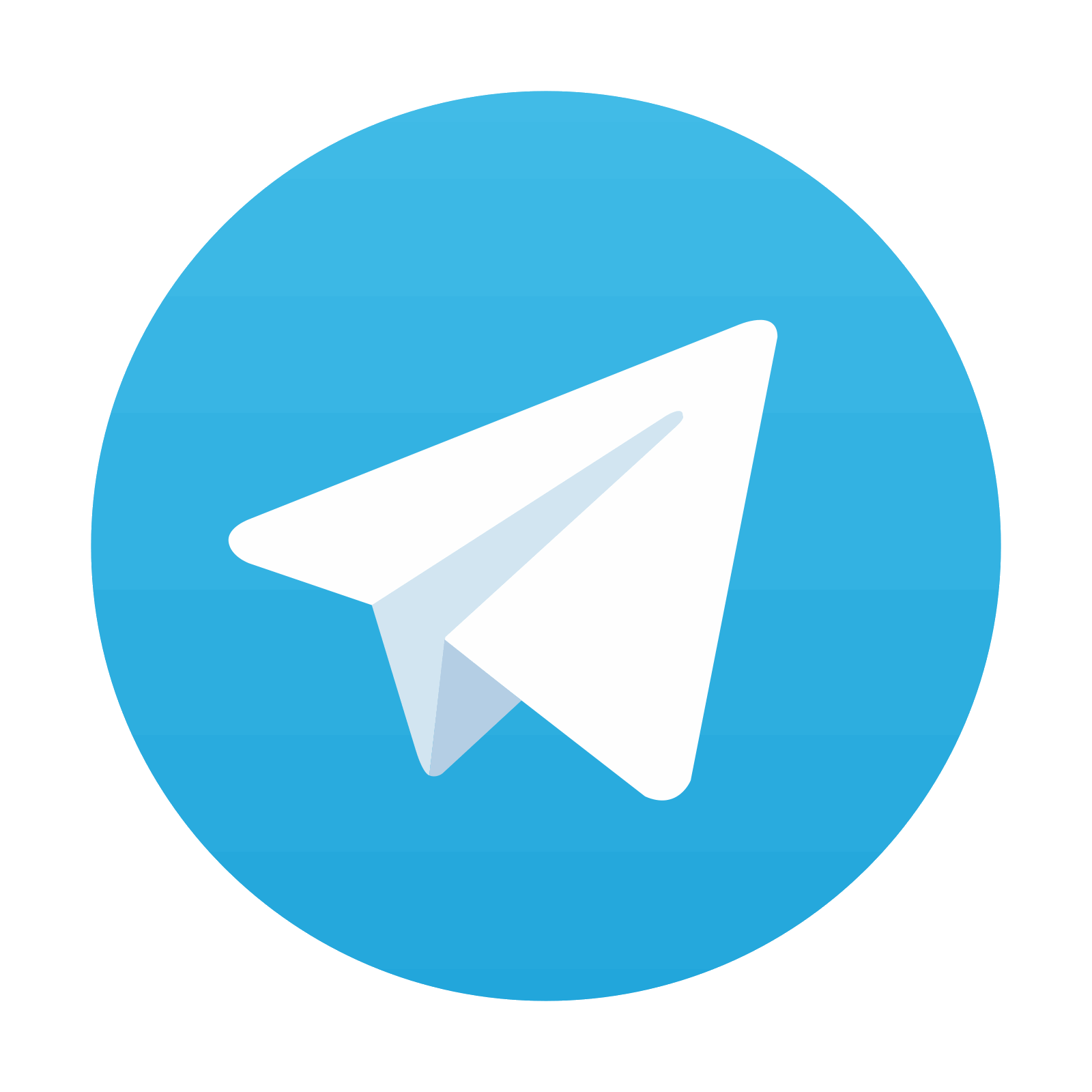
Stay updated, free articles. Join our Telegram channel
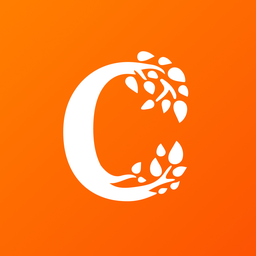
Full access? Get Clinical Tree
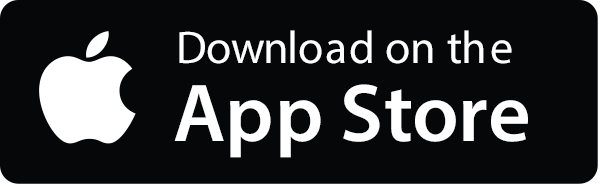
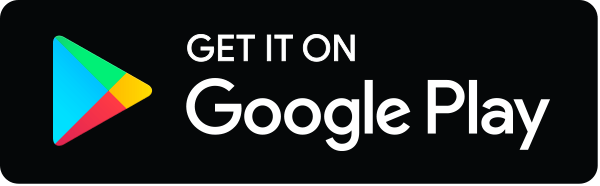