Objective
Microbial invasion of the amniotic cavity (MIAC) is common in early preterm labor and is associated with maternal and neonatal infectious morbidity. MIAC is usually occult and is reliably detected only with amniocentesis. We sought to develop a noninvasive test to predict MIAC based on protein biomarkers in cervicovaginal fluid (CVF) in a cohort of women with preterm labor (phase 1) and to validate the test in an independent cohort (phase 2).
Study Design
This was a prospective study of women with preterm labor who had amniocentesis to screen for MIAC. MIAC was defined by positive culture and/or 16S ribosomal DNA results. Nine candidate CVF proteins were analyzed by enzyme-linked immunosorbent assay. Logistic regression was used to identify combinations of up to 3 proteins that could accurately classify the phase 1 cohort (N = 108) into those with or without MIAC. The best models, selected by area under the curve (AUC) of the receiver operating characteristic curve in phase 1, included various combinations of interleukin (IL)-6, chemokine (C-X-C motif) ligand 1 (CXCL1), alpha fetoprotein, and insulin-like growth factor binding protein-1. Model performance was then tested in the phase 2 cohort (N = 306).
Results
MIAC was present in 15% of cases in phase 1 and 9% in phase 2. A 3-marker CVF model using IL-6 plus CXCL1 plus insulin-like growth factor binding protein-1 had AUC 0.87 in phase 1 and 0.78 in phase 2. Two-marker models using IL-6 plus CXCL1 or alpha fetoprotein plus CXCL1 performed similarly in phase 2 (AUC 0.78 and 0.75, respectively), but were not superior to CVF IL-6 alone (AUC 0.80). A cutoff value of CVF IL-6 ≥463 pg/mL (which had 81% sensitivity in phase 1) predicted MIAC in phase 2 with sensitivity 79%, specificity 78%, positive predictive value 38%, and negative predictive value 97%.
Conclusion
High levels of IL-6 in CVF are strongly associated with MIAC. If developed into a bedside test or rapid laboratory assay, cervicovaginal IL-6 might be useful in selecting patients in whom the probability of MIAC is high enough to warrant amniocentesis or transfer to a higher level of care. Such a test might also guide selection of potential subjects for treatment trials.
Intraamniotic infection and inflammation are frequent causes of preterm labor, especially at early gestational ages. Preterm labor accompanied by infection and/or inflammation has a worse prognosis than preterm labor without infection or inflammation, including high rates of failed tocolysis, delivery within 48 hours, and neonatal morbidity and mortality. Microbial invasion of the amniotic cavity (MIAC) is the most common identified cause of intraamniotic inflammation.
In principle, the optimal management of preterm labor may be different for those with MIAC than for those without. For example, we would hypothesize that antibiotics, corticosteroids, and other antiinflammatory medications may be differentially effective depending on the presence or absence of infection. However, this is speculative because there is currently not a practical, reliable way to rapidly diagnose MIAC. Rapid detection is essential because most patients with MIAC deliver within a day or 2 of presentation.
Most cases of MIAC do not have classic signs of chorioamnionitis such as fever, uterine tenderness, or tachycardia. Often, preterm labor itself is the only manifestation of MIAC. Currently, accurate diagnosis of MIAC depends on analysis of amniotic fluid obtained via amniocentesis. But many patients and physicians are reluctant to perform amniocentesis because of concern that the procedure may cause rupture of the membranes, bleeding, or iatrogenic infection. Even if amniocentesis is performed, the currently available tests do not give rapid diagnostic information. Amniotic fluid Gram stain is relatively insensitive and glucose testing is nonspecific ; amniotic fluid white cell count is nonspecific and can be falsely elevated by blood, which is detectable in significant amounts in >20% of specimens. Culture was previously regarded as the gold standard for diagnosing infection but results can take several days. Moreover, many fastidious organisms fail to grow in culture but can be detected by DNA-based testing. A rapid polymerase chain reaction technique is being developed that might allow timely detection of bacterial and fungal DNA in amniotic fluid, but this is not widely available at hospital laboratories.
Intraamniotic infection and inflammation are associated with changes in the concentrations of several proteins in cervicovaginal fluid (CVF). Prior investigators have suggested that a test using protein biomarkers in CVF could have adequate sensitivity and specificity for detection of MIAC or intraamniotic inflammation or for prediction of preterm birth. In these studies, however, the performance of each proposed test was evaluated in the same subject group from which the models were derived. For validation, confirmation in an independent group is required.
The goal of the present investigation was to develop a noninvasive test to detect MIAC using concentrations of protein biomarkers in CVF. The models were developed in 1 group of women in preterm labor and then the diagnostic performance of the models was tested in a second group.
Materials and Methods
This is the primary analysis of a prospective, multicenter study conducted at 23 tertiary-care perinatal centers across the United States. The sites are listed in the “Acknowledgments” section. The protocol was approved at the institutional review board at each site. All participants gave written, informed consent to participate. The study was registered on clinicaltrials.gov , identifier NCT00700219 . Details of some of the methods have been reported previously.
The study was conducted in 3 phases. Phase 1 included subjects enrolled from September 2007 through May 2008 and was used for preliminary selection of CVF proteins to target for detailed analysis and for development of multiprotein models to predict MIAC. Phase 2 included subjects enrolled from July 2008 through November 2009 and was used to test the diagnostic performance of the multiprotein models. Phase 3 included subjects enrolled from November 2009 through August 2010. The CVF specimens from phase 3 are being held in a biobank at –70°C and have not yet been analyzed, so phase 3 subjects are not included in the present report.
Inclusion criteria were: singleton pregnancy, preterm labor with intact membranes at 22 0/7 to 36 6/7 weeks of gestation, maternal age ≥18 years, and amniocentesis performed to evaluate for intraamniotic infection. Preterm labor was defined as regular uterine contractions plus at least one of the following indicators of cervical change: cervical dilation ≥2 cm, cervical length by transvaginal sonogram ≤30 mm, or positive fetal fibronectin (FFN) test from cervicovaginal secretions. All study sites routinely offered amniocentesis to women in preterm labor as part of their standard management. Exclusion criteria were ruptured membranes, major fetal anomaly, fetal aneuploidy, or a medical indication for preterm birth. Inclusion criteria for phase 1 were broader than for phases 2 and 3, including gestational age as low as 15 0/7 weeks and exceptions to the requirement for amniocentesis in certain circumstances. For the present report, we included phase 1 subjects only if they met all of the more stringent criteria used in phases 2 and 3.
CVF was obtained and processed as described previously. Briefly, during a speculum examination a polyester swab was placed in the posterior vaginal fornix for 20 seconds. Following collection, protein was extracted into 2 mL of transport media (phosphate-buffered saline containing a protease inhibitor cocktail; Roche Diagnostics, Alameda, CA). Samples were spun after extraction to remove any debris and cellular material, and the supernatant was stored at –70°C until analysis.
Amniotic fluid was obtained via transabdominal amniocentesis using aseptic technique and sonographic guidance. A 5-mL aliquot was sent to the local hospital laboratory for aerobic and anaerobic culture and a 10-mL aliquot was frozen and sent to the ProteoGenix Inc (Costa Mesa, CA) central laboratory. Cultures were performed at reference laboratories (University of Washington, Seattle, WA, for phase 1; Focus Diagnostics, Cypress, CA, for phase 2) using broth enrichment techniques to detect low levels of aerobic and anaerobic bacteria, including genital mycoplasmas, as described elsewhere. The polymerase chain reaction and 16S ribosomal DNA (rDNA) procedures were performed as detailed previously. Culture and 16S rDNA results were reviewed and confirmed by a consultant microbiologist. MIAC was defined as a positive culture and/or a positive 16S rDNA. Amniotic fluid interleukin (IL)-6 concentration was measured with a commercial kit following the manufacturer’s instructions (Quantikine enzyme-linked immunosorbent assay [ELISA] #D6050; R&D Systems, Minneapolis, MN). In phase 1, amniotic fluid IL-6 was assayed for all specimens. In phase 2, the study sponsor elected to suspend measurement of amniotic fluid IL-6 after 192 of the 306 specimens had been analyzed.
Other than specimen collection (CVF, amniotic fluid, serum, urine, placenta, as described previously ), the remainder of the clinical care was at the discretion of the managing clinicians, including decisions such as tocolysis, antenatal corticosteroids, and timing and route of delivery.
The first step in biomarker discovery was an exploration of 43 candidate CVF proteins in specimens from phase 1, including cytokines, chemokines, and other inflammatory markers. These were assayed by ELISA using house-developed tests at ProteoGenix or using a commercial laboratory (Rules-Based Medicine Inc, Austin, TX). For each candidate protein a receiver operating characteristic (ROC) curve was generated and area under the curve (AUC) was used to summarize the association between protein biomarker concentration and intraamniotic infection and/or inflammation, defined as MIAC and/or amniotic fluid IL-6 ≥11 ng/mL. From the list of 43 candidate CVF proteins, 9 were selected for targeted analysis, based on a variety of criteria such as AUC, robustness, independence (ie, lack of correlation with other markers), biologic plausibility, simplicity of the assay, and patent considerations. We also favored proteins in which AUC was relatively uninfluenced by the presence of blood in the specimen (analyzed by hemoglobin and human serum albumin concentrations).
In phase 2, the 9 targeted proteins were assayed on Proteogenix test kits configured as a quantitative sandwich immunoassay (ELISA). A monoclonal antibody specific for the analyte was bound to individual wells in a polystyrene microtiter strip plate. Supernatant CVF samples, controls, and standards were added and incubated to allow the analyte to bind the specific capture antibody. All assays were performed on 100-μL samples. The wells were washed to remove unbound substances and nonspecific reactants. A biotinylated polyclonal antibody specific for the analyte was then added and incubated to allow the detection antibody to bind to the captured antigen. Following a second wash to remove unbound antibody reagent, a streptavidin-horseradish-peroxidase conjugate was added. Another wash was performed to remove unbound conjugate. A tetramethylbenzidine substrate solution was added and color was allowed to develop. Stop reagent was added and the color change was measured with a microtiter plate photometer at 450 nm. Sample optical density readings were compared with reference standard optical density readings to determine antigen concentrations. These techniques yielded a more sensitive lower limit of detection than the assays used in phase 1. For example, for IL-6, the lower limit of detection was 312 pg/mL in phase 1 and 63 pg/mL in phase 2.
To develop a test for MIAC, we began by considering each of the 9 CVF biomarkers individually and combinations of 2 or 3 biomarkers using phase 1 data. For each biomarker or combination, we optimized the relative weights of each protein via logistic regression. We selected the 4 top-performing models based on 2 criteria. First, we evaluated their ability to distinguish phase 1 subjects with MIAC or without MIAC as measured by AUC. Second, we favored parsimonious models, that is, if 2 models had similar AUC, we favored the model based on fewer biomarkers.
For validation, the 4 top-performing models from phase 1 were tested in the independent phase 2 cohort. We examined the performance of these models with phase 2 data in terms of AUC, sensitivity, specificity, positive and negative predictive values, and positive and negative likelihood ratios.
Statistical analyses were performed with SAS software Version 9.4 (SAS Institute, Cary, NC) and R Version 3.1 base and pROC packages (R Foundation for Statistical Computing, Vienna, Austria). Between-group differences were tested with t test for normal variables or log-transformed normalized variables, U test for nonnormal continuous variables, and χ 2 test for categorical variables. Nonparametric P values were calculated for AUC. Two-tailed P values < .05 were considered significant.
Results
From September 2007 through August 2010, a total of 701 subjects were enrolled, as summarized in Figure 1 . After various exclusions, 414 were analyzed for the present report, including 108 from phase 1 and 306 from phase 2.
Clinical characteristics at presentation are summarized in Table 1 . Mean gestational age at enrollment was slightly lower and median amniotic fluid IL-6 concentration was slightly higher in phase 1 than in phase 2. Otherwise, the presentations were similar in the 2 phases.
Characteristic | Phase 1 (n = 108) | Phase 2 (n = 306) | P value |
---|---|---|---|
Demographic | |||
Maternal age, y a | 26.3 ± 5.5 | 25.8 ± 5.7 | .397 |
Nulliparous b | 31 (29) | 92 (30) | .971 |
Presentation | |||
Gestational age, wk a | 29.0 ± 3.8 | 30.2 ± 4.0 | .007 |
Cervical dilation, cm c | 3.0 (2.0–3.0) | 3.0 (2.0–4.0) | .133 |
Cervical dilation ≥2 cm b | 77 (80) | 190 (81) | .958 |
Amniotic fluid findings | |||
Microbial invasion of amniotic cavity b | 16 (15) | 29 (9.5) | .176 |
Interleukin-6, ng/dL c | 0.9 (0.5–10.3) | 1.8 (1.4–4.0) | < .0001 |
Interleukin-6 ≥11.3 ng/dL b | 26 (24) | 35 (18) | .227 |
The results of the phase 1 biomarker discovery analysis of 43 CVF proteins are summarized in Table 2 . The 9 proteins listed at the top of Table 1 were selected for model building in phase 1 and were the only CVF proteins analyzed during phase 2. The selection of these 9 proteins was based, in part, on the AUC of each protein to predict intraamniotic inflammation (amniotic fluid IL-6 ≥11 ng/mL) and/or MIAC (positive culture and/or 16S rDNA). For model building, however, we were interested only in the AUC for prediction of MIAC, without consideration of amniotic fluid IL-6. The AUC for prediction of MIAC for each of the 9 proteins is tabulated separately in Table 2 . Some proteins (eg, IL-8 and plasminogen activator inhibitor-1) were not selected for model building despite high AUC because they were highly correlated with IL-6 concentration and thus would add little to any models that included IL-6. Chemokine (C-X-C motif) ligand 1 (CXCL1, formerly known as GRO-alpha) was selected for model building despite a low single-marker AUC because it was highly independent of all the other proteins and it contributed to the AUC in multiple-marker models in phase 1. Further, CXCL1 was a negative predictor, ie, intraamniotic infection and/or inflammation were associated with lower CVF concentrations of this protein, whereas all the other selected proteins were positive predictors, averaging higher in the presence of infection/inflammation.
Abbreviation | Names(s) | Putative role | Assay | Exploration based on MIAC and/or AF IL-6 ≥11 ng/mL | Protein concentration in cervicovaginal fluid, pg/mL | Findings based on MIAC only | ||
---|---|---|---|---|---|---|---|---|
AUC | P value | MIAC | No MIAC | AUC (95% CI) | ||||
9 proteins selected for further analysis | ||||||||
IL-6 | Interleukin-6 | Cytokine | PGX | 0.848 | < .001 | 2153 ± 4.3 | 569 ± 2.8 | 0.80 (0.68–0.92) |
α1AG | Alpha 1-acid glycoprotein | Acute phase reactant | PGX | 0.678 | .005 | 1501 ± 11.0 | 879 ± 6.2 | 0.64 (0.48–0.81) |
IGFBP-1 | Insulin-like growth factor binding protein-1 | Binding protein | PGX | 0.744 | < .001 | 991 ± 6.1 | 345 ± 6.7 | 0.66 (0.52–0.79) |
MIP-1β | Macrophage inflammatory protein 1-beta | Chemokine | RBM | 0.762 | < .001 | 763 ± 3.7 | 477 ± 4.3 | 0.62 (0.48–0.77) |
MCP-1 | Monocyte chemotactic protein 1 (chemokine [C-C motif] ligand 2 [CCL2]) | Cytokine | RBM | 0.757 | < .001 | 689 ± 4.5 | 258 ± 3.1 | 0.70 (0.54–0.87) |
LBP | Lipopolysaccharide binding protein | Acute phase reactant | PGX | 0.696 | .001 | 229 ± 2.1 | 140 ± 2.4 | 0.71 (0.59–0.83) |
β2MG | Beta-2 microglobulin | Cell surface protein | RBM | 0.732 | < .001 | 77.0 ± 6.1 | 32.3 ± 3.9 | 0.71 (0.53–0.88) |
AFP | Alpha fetoprotein | Plasma protein | RBM | 0.868 | < .001 | 45.0 ± 6.1 | 9.3 ± 3.0 | 0.74 (0.58–0.90) |
CXCL1 | Chemokine (C-X-C motif) ligand 1, aka growth-regulated protein alpha (GRO-alpha) | Cytokine | PGX | 0.556 | .385 | 2333 ± 1.5 | 3225 ± 2.1 | 0.61 (0.49–0.73) |
34 proteins not explored beyond phase 1 | ||||||||
α1AT | Alpha 1-antitrypsin | Protease inhibitor | RBM | 0.604 | .103 | |||
Adiponectin | Adiponectin | Regulatory hormone | RBM | 0.626 | .051 | |||
APOA-1 | Apolipoprotein A1 | Antiinflammatory protein | RBM | 0.628 | .048 | |||
BP-1 | Beta protein 1 | Inflammatory marker | PGX | 0.709 | .001 | |||
C3 | Complement component 3 | Inflammatory mediator | RBM | 0.600 | .116 | |||
CCL5 | Chemokine (C-C motif) ligand 5, aka regulated on activation normal T-cell expressed and secreted RANTES | Chemokine | RBM | 0.664 | .012 | |||
CEA | Carcinoembryonic antigen | Cell adhesion molecule | RBM | 0.746 | .001 | |||
Ceruloplasmin | Ceruloplasmin | Acute phase reactant | PGX | 0.597 | .123 | |||
CXCL5 | Chemokine (C-X-C motif) ligand 5, aka epithelial-derived neutrophil-activating peptide 78 (ENA-78) | Cytokine | RBM | 0.546 | .455 | |||
FABP | Fatty acid-binding protein | Transport protein | RBM | 0.675 | .007 | |||
Ferritin | Ferritin | Nonspecific inflammatory marker | RBM | 0.718 | < .001 | |||
Fibrinogen | Fibrinogen | Coagulation protein | RBM | 0.629 | .045 | |||
GCSF | Granulocyte-macrophage colony stimulating factor | Cytokine | RBM | 0.676 | .007 | |||
HGF | Hepatocyte growth factor | Growth factor | RBM | 0.741 | < .001 | |||
HNP1-3 | Human neutrophil peptides 1-3, aka alpha defensins 1-3 | Antimicrobial peptides | PGX | 0.732 | < .001 | |||
IL-1β | Interleukin-1 beta | Cytokine | RBM | 0.767 | < .001 | |||
IL-6R | Interleukin-6 receptor | Cytokine receptor | RBM | 0.641 | .029 | |||
IL-8 | Interleukin-8 | Chemokine | RBM | 0.824 | < .001 | |||
Lysozyme | Lysozyme | Immune modulator | PGX | 0.633 | .034 | |||
MMP-7 | Matrix metalloproteinase-7 | Protease | RBM | 0.736 | < .001 | |||
MMP-8 | Matrix metalloproteinase-8 | Protease | PGX | 0.597 | .135 | |||
MPOD | Myeloperoxidase | Inflammatory marker | RBM | 0.676 | .006 | |||
Myoglobin | Myoglobin | Binding protein | RBM | 0.716 | < .001 | |||
PAI-1 | Plasminogen activator inhibitor-1 | Protease inhibitor | RBM | 0.825 | < .001 | |||
PAPP-A | Pregnancy-associated plasma protein-A | Inflammatory marker | RBM | 0.692 | .003 | |||
PRL | Prolactin | Immune modulator | RBM | 0.712 | .001 | |||
S100A12 | Calgranulin C, aka extracellular novel receptor for advanced glycation end products (EN-RAGE) | Inflammatory marker | RBM | 0.714 | .001 | |||
TF | Tissue factor | Inflammatory mediator | RBM | 0.643 | .027 | |||
Thrombin | Thrombin | Clotting factor | RBM | 0.697 | .003 | |||
TIMP-1 | Tissue inhibitor of metalloproteinases-1 | Protease inhibitor | RBM | 0.734 | < .001 | |||
TNFR-2 | Tumor necrosis factor receptor 2 | Binding protein | RBM | 0.720 | < .001 | |||
TRAIL-R3 | Tumor necrosis factor–related apoptosis-inducing ligand receptor 3 | Cytokine receptor | RBM | 0.699 | .002 | |||
VCAM-1 | Vascular cell adhesion molecule 1 (cluster of differentiation 106 [CD106]) | Endothelial protein | RBM | 0.720 | < .001 | |||
VDBP | Vitamin D-binding protein | Binding protein | RBM | 0.662 | .013 |
The ROC curves of the 4 top-performing prediction models from phase 1 are shown in the left panel of Figure 2 . These models were based on the concentrations of 4 CVF proteins: IL-6, alpha-fetoprotein, insulin-like growth factor binding protein-1, and CXCL1. The 4 models involved CVF concentrations of: IL-6 (alone), IL-6 plus CXCL1, alpha-fetoprotein plus CXCL1, and IL-6 plus CXCL1 plus insulin-like growth factor binding protein-1. The AUC for these ranged from 0.80-0.87. The 3 models that included multiple markers all had AUCs that were higher than the AUC for any of the 9 individual markers ( Table 2 ).
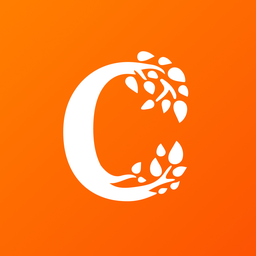
Full access? Get Clinical Tree
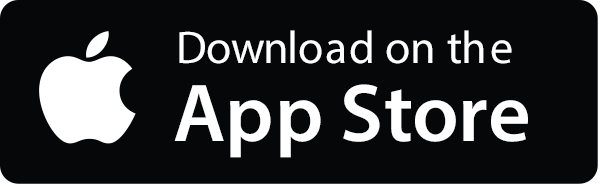
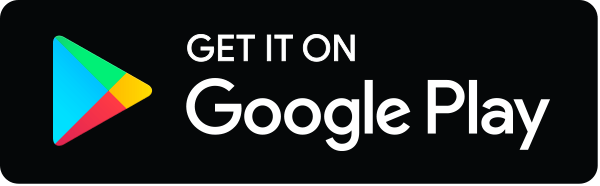