Chapter 80 Defects in Metabolism of Lipids
80.1 Disorders of Mitochondrial Fatty Acid β-Oxidation
Mitochondrial β-oxidation of fatty acids is an essential energy-producing pathway. It is a particularly important pathway during prolonged periods of starvation, and during periods of reduced caloric intake due to gastrointestinal illness or increased energy expenditure during febrile illness. Under these conditions, the body switches from using predominantly carbohydrate to predominantly fat as its major fuel. Fatty acids are also important fuels for exercising skeletal muscle and are the preferred substrate for the heart. In these tissues, fatty acids are completely oxidized to carbon dioxide and water. The end products of hepatic fatty acid oxidation are the ketone bodies β-hydroxybutyrate and acetoacetate. These cannot be oxidized by the liver but serve as important fuels in peripheral tissues, particularly the brain.
Genetic defects have been identified in nearly all of the known steps in the fatty acid oxidation pathway; all are recessively inherited (Table 80-1).
Clinical manifestations characteristically involve the tissues with a high β-oxidation flux including liver, skeletal, and cardiac muscle. The most common presentation is an acute episode of life-threatening coma and hypoglycemia induced by a period of fasting due to defective hepatic ketogenesis. Other manifestations include chronic cardiomyopathy and muscle weakness or exercise-induced acute rhabdomyolysis. The fatty acid oxidation defects can be asymptomatic during periods when there is no fasting stress. Acutely presenting disease may be misdiagnosed as Reye syndrome or, if fatal, as sudden unexpected infant death. Fatty acid oxidation disorders are easily overlooked because the only specific clue to the diagnosis may be the finding of inappropriately low concentrations of urinary ketones in an infant who has hypoglycemia. Genetic defects in ketone body utilization may be overlooked because ketosis is an expected finding with fasting hypoglycemia. In some circumstances, clinical manifestations appear to arise from toxic effects of fatty acid metabolites rather than inadequate energy production. These include disorders (LCHAD, CPT-IA, TFP) in which the presence of a homozygous affected fetus increases the risk of a life-threatening illness in the heterozygote mother, resulting in acute fatty liver of pregnancy or preeclampsia with HELLP (hemolysis, elevated liver enzymes, low platelets) syndrome. Malformations of the brain and kidneys have been described in severe electron transfer flavoprotein (ETF), ETF dehydrogenase (ETF-DH), and carnitine palmitoyltransferase-2 (CPT-II) deficiencies that might reflect in utero toxicity of fatty acid metabolites. Progressive retinal degeneration, peripheral neuropathy and chronic progressive liver disease have been identified in LCHAD and TFP deficiency. Newborn screening programs using tandem mass spectrometry (MS/MS) detect characteristic acylcarnitines seen in many of these disorders and permit presymptomatic diagnosis. Screening programs have provided evidence that all the fatty acid oxidation disorders combined are among the most common inborn errors of metabolism.
Figures 80-1 and 80-2 outline the steps involved in the oxidation of a typical long-chain fatty acid. In the carnitine cycle, fatty acids are transported across the barrier of the inner mitochondrial membrane as acylcarnitine esters. Within the mitochondria, successive turns of the 4-step β-oxidation cycle convert the coenzyme A (CoA)-activated fatty acid to acetyl CoA units. Two to three different chain-length specific isoenzymes are needed for each of these β-oxidation steps to accommodate the different-sized fatty acyl CoA species. The electron transfer pathway carries electrons generated in the 1st β-oxidation step (acyl CoA dehydrogenase) to the electron transport chain for adenosine triphosphate (ATP) production, while electrons generated from the third step (3-hydroxyacyl CoA dehydrogenase) enter the respiratory chain at the level of complex 1. Most of the acetyl CoA generated from hepatic β-oxidation flows through the pathway of ketogenesis to form β-hydroxybutyrate and acetoacetate.
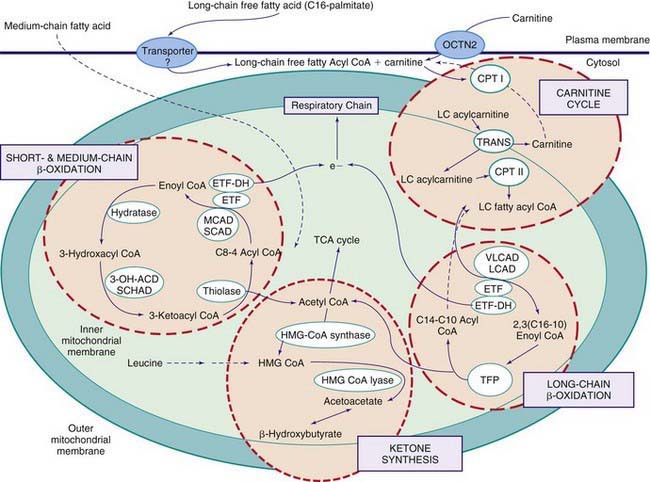
Figure 80-1 Mitochondrial fatty acid oxidation. Carnitine enters the cell through the action of the organic cation/carnitine transporter (OCTN2). Palmitate, a typical 16-carbon long-chain fatty acid, is transported across the plasma membrane and can be activated to form a long-chain (LC) fatty acyl coenzyme A (CoA). It then enters into the carnitine cycle, where it is transesterified by carnitine palmitoyltransferase-I (CPT-I), translocated across the inner mitochondrial membrane by carnitine/acylcarnitine translocase (TRANS), and then reconverted into a long-chain fatty acyl CoA by carnitine palmitoyltransferase-II (CPT-II) to undergo β-oxidation. Very long chain acyl CoA dehydrogenase (VLCAD/LCAD) leads to the production of (C16-10) 2,3 enoyl CoA. Trifunctional protein (TFP) contains the activities of enoyl CoA hydratase (hydratase), 3-OH-hydroxyacyl CoA dehydrogenase (3-OH-ACD), and β-ketothiolase (thiolase). Acetyl CoA, FADH, and NADH are produced. Medium- and short-chain fatty acids (C8-4) can enter the mitochondrial matrix independent of the carnitine cycle. Medium-chain acyl CoA dehydrogenase (MCAD), short-chain acyl CoA dehydrogenase (SCAD), and short-chain hydroxy acyl CoA dehydrogenase (SCHAD) are required. Acetyl CoA can then enter the Krebs (TCA) cycle. Electrons are transported from FADH to the respiratory chain via the electron transfer flavoprotein (ETF) and the electron transfer flavoprotein dehydrogenase (ETF-DH). NADH enters the electron transport chain through complex I. Acetyl CoA can be converted into hydroxymethylglutaryl (HMG) CoA by β-hydroxy-β-methylglutaryl CoA synthase (HMG CoA synthase) and then the ketone body acetoacetate by the action of β-hydroxy-β-methylglutaryl CoA lyase (HMG CoA lyase).
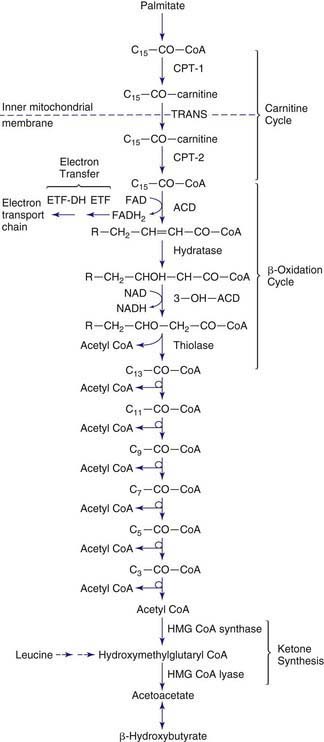
Figure 80-2 Pathway of mitochondrial oxidation of palmitate, a typical 16-carbon long-chain fatty acid. Enzyme steps include carnitine palmitoyltransferase (CPT) 1 and 2, carnitine/acylcarnitine translocase (TRANS), electron transfer flavoprotein (ETF), ETF dehydrogenase (ETF-DH), acyl CoA dehydrogenase (ACD), enoyl CoA hydratase (hydratase), 3-hydroxy-acyl CoA dehydrogenase (3-OH-ACD), β-ketothiolase (thiolase), β-hydroxy-β-methylglutaryl CoA (HMG CoA) synthase, and lyase.
Defects in the β-Oxidation Cycle
Medium-Chain Acyl CoA Dehydrogenase (MCAD) Deficiency
MCAD deficiency is the most common fatty acid oxidation disorder. The disorder shows a strong founder effect; most patients have a northwestern European ancestry, and the majority of patients are homozygous for a single common missense mutation, an A-G transition at cDNA position 985 that changes a lysine to glutamic acid at residue 329 (K329E).
Clinical Manifestations
Affected patients usually present in the 1st 3 mo-5 yr of life with episodes of acute illness triggered by prolonged fasting (longer than 12-16 hr). Signs and symptoms include vomiting and lethargy, which rapidly progress to coma or seizures and cardiorespiratory collapse. Sudden unexpected infant death may occur. The liver may be slightly enlarged with fat deposition. Attacks are rare until the infant is beyond the 1st few months of life, presumably due to more frequent feedings at a younger age. Affected older infants are at higher risk of illness as they begin to fast through the night or are exposed to fasting stress during an intercurrent childhood illness. Presentation in the 1st days of life with neonatal hypoglycemia, has been reported in newborns that were fasted inadvertently. Diagnosis of MCAD has occasionally been documented in previously healthy teenage and adult individuals, indicating that even patients who have been asymptomatic in infancy are still at risk for metabolic decompensation if exposed to sufficient periods of fasting. An unknown number may remain asymptomatic.
Laboratory Findings
During acute episodes, hypoglycemia is usually present. Plasma and urinary ketone concentrations are inappropriately low (hypoketotic hypoglycemia). Because of the relative hypoketonemia, there is little or no metabolic acidemia. Tests of liver function are abnormal, with elevations of liver enzymes (ALT, AST), elevated blood ammonia, and prolonged prothrombin (PT) and partial thromboplastin times (PTT). Liver biopsy at times of acute illness shows microvesicular or macrovesicular steatosis due to triglyceride accumulation. During fasting stress or at times of acute illness, urinary organic acid profiles by gas chromatography/mass spectrometry show inappropriately low concentrations of ketones and elevated levels of medium-chain dicarboxylic acids (adipic, suberic, and sebacic acids) that derive from microsomal and peroxisomal omega oxidation of fatty acids. Plasma and tissue concentrations of total carnitine are reduced to 25-50% of normal, and the fraction of total esterified carnitine is increased. This pattern of secondary carnitine deficiency is seen in most fatty acid oxidation defects and reflects competition between increased acylcarnitine levels and free carnitine transport at the plasma membrane. Significant exceptions to this rule are the plasma membrane carnitine transporter, CPT-IA and β-hydroxy-β-methylglutaryl CoA (HMG CoA) synthase deficiencies.
Diagnostic patterns include increased plasma C8:0, C10:0, and C10:1 acylcarnitine species and increased urinary acylglycines including hexanoyl-, suberyl- and 3-phenylpropionyl glycines. Newborn screening programs using tandem mass spectrometry which almost all babies born in the USA receive can diagnose presymptomatic MCAD deficiency based on the detection of the abnormal acylcarnitines in filter paper blood spots. In many cases, the diagnosis can be confirmed by finding the common A985G mutation. A second common variant, T199C, has been detected in infants with characteristic acylcarnitines in newborn screening tests. Interestingly, this allele has not been seen to date in symptomatic MCAD patients; it may represent a mild mutation.
Treatment
Acute illnesses should be promptly treated with intravenous fluids containing 10% dextrose to treat or prevent hypoglycemia and to suppress lipolysis as rapidly as possible (Chapter 86). Chronic therapy consists of avoiding fasting. This usually requires simply adjusting the diet to ensure that overnight fasting periods are limited to <10-12 hr. Restricting dietary fat or treatment with carnitine is controversial. The necessity for active therapeutic intervention for individuals with the T199C variant has not yet been established.
Prognosis
Up to 25% of unrecognized patients may die during their 1st attack of illness. There is frequently a history of a previous sibling death due to unrecognized MCAD deficiency. Some patients may develop permanent brain injury during an attack of profound hypoglycemia. The prognosis for survivors without brain damage is excellent because cognitive impairment or cardiomyopathy does not occur in MCAD deficiency. Muscle pain and reduced exercise tolerance may become evident with increasing age. Fasting tolerance improves with age and the risk of illness decreases. As many as 35% of affected patients have never had an episode; therefore, testing of siblings of affected patients is important to detect asymptomatic family members.
Very Long Chain Acyl CoA Dehydrogenase (VLCAD) Deficiency
VLCAD deficiency is the second most commonly diagnosed disorder of fatty acid oxidation. It was originally termed LCAD deficiency before the existence of the inner mitochondrial membrane-bound VLCAD was known. All patients previously diagnosed as having LCAD deficiency have VLCAD enzyme deficiency. Patients with VLCAD deficiency are usually more severely affected than those with MCAD deficiency, presenting earlier in infancy and having more chronic problems with muscle weakness or episodes of muscle pain and rhabdomyolysis. Cardiomyopathy may be present during acute attacks associated with fasting. The left ventricle may be hypertrophic or dilated and show poor contractility on echocardiography. Sudden unexpected death has occurred in several patients, but most who survived the initial episode showed improvement, including normalization of cardiac function. Other physical and routine laboratory features are similar to those of MCAD deficiency, including secondary carnitine deficiency. The urinary organic acid profile shows a nonketotic dicarboxylic aciduria. Increased levels of C6-12 dicarboxylic acids may be noted in the urine. Diagnosis may be suggested by an abnormal acylcarnitine profile with plasma or blood spot C14:1,14:0 acylcarnitine species, but the specific diagnosis requires assay of enzyme activities of VLCAD in cultured fibroblasts or direct mutational analysis of the VLCAD gene. Treatment is based primarily on avoidance of fasts for >10-12 hr. Continuous intragastric feeding is useful in some patients.
Short-Chain Acyl CoA Dehydrogenase (SCAD) Deficiency
A small number of patients with two clear null mutations in the SCAD gene have been described with variable phenotype. Most individuals classified as being SCAD deficient have been shown to have polymorphic DNA changes in the SCAD gene. The two common polymorphisms are G185S and R147W, which are present in 7% of the population. Some investigators argue that these may be susceptibility changes, which require a second, as yet unknown, genetic mutation to express a clinical phenotype; while others believe that SCAD deficiency is a harmless biochemical condition. This autosomal recessive disorder presents with neonatal hypoglycemia and may have normal levels of ketone bodies. The diagnosis is indicated by elevated levels of butyrylcarnitine on blood spots or plasma and increased excretion of urinary ethylmalonic acid and butyrylglycine. These metabolic abnormalities are most pronounced in patients with null mutations and variably present in patients who are homozygous for the polymorphisms.
The necessity for treatment in SCAD deficiency has not yet been established. It has been proposed that long-term evaluation of symptomless individuals is necessary to determine whether this is or is not a real disease.
Long-Chain 3-Hydroxyacyl CoA Dehydrogenase (LCHAD)/Mitochondrial Trifunctional Protein (TFP) Deficiency
The LCHAD enzyme is part of a mitochondrial trifunctional protein (TFP), which also contains two other steps in β-oxidation, long-chain enoyl CoA hydratase and long-chain β-ketothiolase. It is a hetero-octameric protein composed of 4 α and 4 β chains that derive from distinct contiguous genes with a common promoter region. In some patients, only the LCHAD activity of the TFP is affected (LCHAD deficiency), whereas others have deficiencies of all 3 activities (TFP deficiency).
Clinical manifestations include attacks of acute hypoketotic hypoglycemia similar to MCAD deficiency; patients often show evidence of more severe disease, including cardiomyopathy, muscle cramps and weakness, and abnormal liver function (cholestasis). Toxic effects of fatty acid metabolites may produce pigmented retinopathy, progressive liver failure, peripheral neuropathy, and rhabdomyolysis. Life-threatening obstetric complications; acute fatty liver of pregnancy; and hemolysis, elevated liver enzymes, low platelets (HELLP) syndrome are observed in heterozygous mothers carrying homozygotic fetuses affected with LCHAD/TFP deficiency. Sudden unexpected infant death may occur. The diagnosis is indicated by elevated levels of blood spot or plasma 3-hydroxy acylcarnitines of chain lengths C16-C18. Urinary organic acid profile in patients may show increases in levels of 3-hydroxydicarboxylic acids of chain lengths C6-C14. Secondary carnitine deficiency is common. A common mutation in the α subunit, E474Q, is seen in >60% of LCHAD deficient patients. This mutation in the fetus is significantly associated with the obstetric complications, but other mutations in either subunit may also be associated with maternal illness.
Treatment is similar to that for MCAD or VLCAD deficiency; that is, avoiding fasting stress. Some investigators have suggested that dietary supplements with medium-chain triglyceride oil to by-pass the long-chain fatty acid oxidation process for long-chain defects and docosahexaenoic acid (DHA, for protection against the retinal changes) may be useful. Liver transplantation does not ameliorate the metabolic abnormalities.
Short-Chain 3-Hydroxyacyl-CoA Dehydrogenase (SCHAD) Deficiency
Very few patients with this inborn error have been described. Only 5 patients with proven mutations of SCHAD have been reported although a few additional unpublished cases are known to the authors. Four cases in 3 families with recessive mutations of SCHAD presented with episodes of hypoketotic hypoglycemia that was shown to be due to hyperinsulinism. In contrast to patients with other forms of fatty acid oxidation disorders, these cases required specific therapy with diazoxide for hyperinsulinism to avoid recurrent hypoglycemia. The 5th child with a mutation was compound heterozygous for two different SCHAD mutations and presented with fulminant hepatic failure at age 10 mo. Other reports of cases not proven to have mutations include a child with attacks of fasting hypoglycemia and myoglobinuria associated with deficiency of SCHAD in muscle but not in cultured fibroblasts, 3 children with fatal liver disease, and an infant who died suddenly and unexpectedly. Specific metabolic markers for SCHAD deficiency include elevated C4-hydroxy acylcarnitine and urine 3-hydroxyglutaric acid.
Treatment of SCHAD deficient patients with hyperinsulinism is with diazoxide.
Defects in the Carnitine Cycle
Plasma Membrane Carnitine Transport Defect (Primary Carnitine Deficiency)
Primary carnitine deficiency is the only genetic defect in which carnitine deficiency is the cause, rather than the consequence, of impaired fatty acid oxidation. The most common presentation is progressive cardiomyopathy with or without skeletal muscle weakness beginning at 1-4 yr of age. A smaller number of patients may present with fasting hypoketotic hypoglycemia in the 1st yr of life before the cardiomyopathy becomes symptomatic. The underlying defect involves the plasma membrane sodium gradient-dependent carnitine transporter that is present in heart, muscle, and kidney. This transporter is responsible both for maintaining intracellular carnitine concentrations 20- to 50-fold higher than plasma concentrations and for renal conservation of carnitine.
Diagnosis of the carnitine transporter defect is aided by the fact that patients have extremely reduced carnitine levels in plasma and muscle (1-2% of normal). Heterozygote parents have plasma carnitine levels approximately 50% of normal. Fasting ketogenesis may be normal because liver carnitine transport is normal, but it may be impaired if dietary carnitine intake is interrupted. The fasting urinary organic acid profile may show a hypoketotic dicarboxylic aciduria pattern if hepatic fatty acid oxidation is impaired, but it is otherwise unremarkable. The defect in carnitine transport can be demonstrated clinically by severe reduction in renal carnitine threshold or in vitro by assay of carnitine uptake using cultured fibroblasts or lymphoblasts. Mutations in the organic cation/carnitine transporter (OCTN2) underlie this disorder. Treatment of this disorder with pharmacologic doses of oral carnitine (100-200 mg/kg/day) is highly effective in correcting the cardiomyopathy and muscle weakness as well as any impairment in fasting ketogenesis. Muscle total carnitine concentrations remain <5% of normal on treatment.
Carnitine Palmitoyltransferase-IA (CPT-IA) Deficiency
Several dozen infants and children have been described with a deficiency of the liver and kidney isozyme of CPT-IA. Clinical manifestations include fasting hypoketotic hypoglycemia, occasionally with markedly abnormal liver function tests and, rarely, with renal tubular acidosis. The heart and skeletal muscle are not involved because the muscle isozyme is unaffected. Fasting urinary organic acid profile shows a hypoketotic C6-C12 dicarboxylic aciduria but may be normal. Plasma acylcarnitine analysis demonstrates mostly free carnitine with very little acylated carnitine. This observation has been used to establish CPT-IA diagnosis on newborn screening by tandem mass spectrometry. CPT-IA deficiency is the only fatty acid oxidation disorder in which plasma total carnitine levels are elevated to 150-200% of normal. This may be explained by the fact that the inhibitory effects of long-chain acylcarnitines on the renal tubular carnitine transporter are absent in CPT-IA deficiency. The enzyme defect can be demonstrated in cultured fibroblasts or lymphoblasts. CPT-IA deficiency in the fetus has been associated with acute fatty liver of pregnancy in the mother in a single case report. A common variant in the CPT1A gene has been identified in individuals of Inuit background in the USA and First Nations tribes in Canada. The variant results in a positive newborn screen and 20% residual enzyme activity which is unregulated. It has not been established if this is a pathological DNA variant or an adaptive process to ancient Inuit and First Nations lifestyles. Treatment for the severe CPT1A deficiency is similar to that for MCAD deficiency with avoidance of situations where fasting ketogenesis is necessary.
Carnitine-Acylcarnitine Translocase (CACT) Deficiency
This defect of the inner mitochondrial membrane carrier protein for fatty acylcarnitines blocks the entry of long-chain fatty acids into the mitochondria for oxidation. The clinical phenotype of this disorder is characterized by a severe and generalized impairment of fatty acid oxidation. Most newborn patients present with attacks of fasting-induced hypoglycemia, hyperammonemia, and cardiorespiratory collapse. All symptomatic newborns have had evidence of cardiomyopathy and muscle weakness. Several patients with a partial translocase deficiency and milder disease without cardiac involvement have also been identified. No distinctive urinary or plasma organic acids are noted, although increased levels of plasma long-chain acylcarnitines are reported. Diagnosis can be made using cultured fibroblasts or lymphoblasts. The human gene has been cloned, and mutations have been identified in affected patients. Treatment is similar to that of other long-chain fatty acid oxidation disorders.
Carnitine Palmitoyltransferase-II (CPT-II) Deficiency
Three forms of CPT-II deficiency have been described. The antenatal presentation of this disorder is associated with a profound enzyme deficiency, and neonatal death has been reported in several newborns with dysplastic kidneys, cerebral malformations, and mild facial anomalies. A severe deficiency of enzyme activity is associated with an infantile-onset form. This form shares all the clinical and laboratory features of CACT deficiency. A milder defect is associated with an adult presentation of episodic rhabdomyolysis. The 1st episode usually does not occur until late childhood or early adulthood. Attacks may be precipitated by prolonged exercise. There is aching muscle pain and myoglobinuria that may be severe enough to cause renal failure. Serum levels of creatine kinase are elevated to 5,000-100,000 U/L. Fasting hypoglycemia has not been described, but fasting may contribute to attacks of myoglobinuria. Muscle biopsy shows increased deposition of neutral fat. The myopathic presentation of CPT-II deficiency is associated with a common mutation S113L. This mutation produces a heat-labile protein that is unstable to increased muscle temperature due to exercise resulting in the myopathic presentation. An intermediate form of CPT-II deficiency presents in infancy/early childhood with fasting-induced hepatic failure, cardiomyopathy, and skeletal myopathy with hypoketotic hypoglycemia but does not have the severe developmental changes seen in the neonatal presentation. This pattern is more like that seen in VLCAD deficiency and management is identical. Patients are generally heterozygous for one of the severe mutations and one of the milder mutations.
Diagnosis of all forms of CPT-II deficiency can be made by demonstrating deficient enzyme activity in muscle or other tissues and in cultured fibroblasts. Mutation analysis is available.
Defects in Electron Transfer Pathway
Electron Transfer Flavoprotein (ETF) and Electron Transfer Flavoprotein Dehydrogenase (ETF-DH) Deficiencies (GLUTARIC Aciduria Type 2, Multiple Acyl CoA Dehydrogenation Deficiencies)
ETF and ETF-DH function to transfer electrons into the mitochondrial electron transport chain from dehydrogenation reactions catalyzed by VLCAD, MCAD, and SCAD, as well as glutaryl CoA dehydrogenase and at least four enzymes involved in branch-chain amino acid oxidation. Deficiencies of ETF or ETF-DH produce illness that combines the features of impaired fatty acid oxidation and impaired oxidation of several amino acids. Complete deficiencies of either protein are associated with severe illness in the newborn period, characterized by acidosis, hypoglycemia, coma, hypotonia, cardiomyopathy, and an unusual odor of sweaty feet due to isovaleryl CoA dehydrogenase inhibition. Some affected neonates have had facial dysmorphism and polycystic kidneys similar to that seen in severe CPT-II deficiency, which suggests that toxic effects of accumulated metabolites may occur in utero.
Diagnosis can be made from the urinary organic acid profile, which shows abnormalities corresponding to blocks in oxidation of fatty acids (ethylmalonate and C6-C10 dicarboxylic acids), lysine (glutarate), and branched-chain amino acids (isovaleryl-, isobutyryl-, and α-methylbutyryl-glycine). Most severely affected infants do not survive the neonatal period.
Partial deficiencies of ETF and ETF-DH produce a disorder that may mimic MCAD deficiency or other milder fatty acid oxidation defects. These patients have attacks of fasting hypoketotic coma. The urinary organic acid profile reveals primarily elevations of dicarboxylic acids and ethylmalonate, derived from short-chain fatty acid intermediates. Secondary carnitine deficiency is present. Some patients with mild forms of ETF/ETF-DH deficiency benefit from treatment with high doses of riboflavin, which is a cofactor for the pathway of electron transfer.
Defects in Ketone Synthesis Pathway
Defects in Ketone Utilization
The ketones β-hydroxybutyrate and acetoacetate are the end products of hepatic fatty acid oxidation and are important as metabolic fuels for the brain during fasting. Two defects in utilization of ketones in brain and other peripheral tissues present as episodes of “hyperketotic” coma, with or without hypoglycemia.
De Leon DD, Stanley CA. Mechanisms of disease: advances in diagnosis and treatment of hyperinsulinism in neonates. Nat Clin Pract Endocrinol Metab. 2007;3:57-68.
Gillingham MB, Weleber RG, Neuringer M, et al. Effect of optimal dietary therapy upon visual function in children with long-chain 3-hydroxyacyl CoA dehydrogenase and trifunctional protein deficiency. Mol Genet Metab. 2005;86:124-133.
Greenberg CR, Dilling LA, Thompson GR, et al. The paradox of the carnitine palmitoyltransferase type 1a P479L variant in Canadian Aboriginal populations. Mol Genet Metab. 2009;96:201-207.
Hsu HW, Zytkovicz TH, Comeau AM, et al. Spectrum of medium-chain acyl-CoA dehydrogenase deficiency detected by newborn screening. Pediatrics. 2008;121:e1108-e1114.
Jethva R, Bennett MJ, Vockley J. Short-chain acyl-coenzyme A dehydrogenase deficiency. Mol Genet Metab. 2008;95:195-200.
Longo N, di San Filippo CA, Pasquali M. Disorders of carnitine transport and the carnitine cycle. Am J Med Genet C Semin Med Genet. 2006;142C:77-85.
Loughrey C, Bennett MJ. Screening for MCAD deficiency in newborns. BMJ. 2009;338:843-846.
Molven A, Matre GE, Duran M, et al. Familial hyperinsulinemic hypoglycemia caused by a defect in the SCHAD enzyme of mitochondrial fatty acid oxidation. Diabetes. 2004;53:221-227.
Schulze A, Matern D, Hoffmann GF. Newborn Screening. In: Sarafoglou K, Hoffmann GF, Roth KS, editors. Pediatric endocrinology and inborn errors of metabolism. New York: McGraw-Hill; 2009:17-32.
Shekhawat PS, Matern D, Strauss AW. Fetal fatty acid oxidation disorders, their effect on maternal health and neonatal outcome: impact of expanded newborn screening on their diagnosis and management. Pediatr Res. 2005;57:78R-86R.
Strauss AW, Andresen BS, Bennett MJ. Mitochondrial fatty acid oxidation defects. In: Sarafoglou K, Hoffmann GF, Roth KS, editors. Pediatric endocrinology and inborn errors of metabolism. New York: McGraw-Hill; 2009:51-70.
van Maldegem BT, Duran M, Wanders RJA, et al. Fasting and fat-loading tests provide pathophysiological insight into short-chain acyl-coenzyme A dehydrogenase deficiency. J Pediatr. 2010;156:121-127.
Wilcken B, Haas M, Joy P, et al. Outcome of neonatal screening for medium-chain acyl-CoA dehydrogenase deficiency in Australia: a cohort study. Lancet. 2007;369:37-42.
80.2 Disorders of Very Long Chain Fatty Acids
Peroxisomal Disorders
The peroxisomal diseases are genetically determined disorders caused either by the failure to form or maintain the peroxisome or by a defect in the function of a single enzyme that is normally located in this organelle. These disorders cause serious disability in childhood and occur more frequently and present a wider range of phenotype than has been recognized in the past.
Etiology
Peroxisomal disorders are subdivided into 2 major categories (Table 80-2).
Table 80-2 CLASSIFICATION OF PEROXISOMAL DISORDERS
A: DISORDERS OF PEROXISOME IMPORT
A1: Zellweger syndrome
A2: Neonatal adrenoleukodystrophy
A3: Infantile Refsum disease
A4: Rhizomelic chondrodysplasia punctata
B: DEFECTS OF SINGLE PEROXISOMAL ENZYME
B1: X-linked adrenoleukodystrophy
B2: Acyl CoA oxidase deficiency
B3: Bifunctional enzyme deficiency
B4: Peroxisomal thiolase deficiency
B5: Classic Refsum disease
B6: 2-Methylacyl CoA racemase deficiency
B7: DHAP acyltransferase deficiency
B8: Alkyl-DHAP synthase deficiency
B9: Mevalonic aciduria
B10: Glutaric aciduria type III
B11: Hyperoxaluria type I
B12: Acatalasemia
In category A, the peroxisomal biogenesis disorders (PBD), the basic defect is the failure to import one or more proteins into the organelle. In category B, defects affect a single peroxisomal protein. The peroxisome is present in all cells except mature erythrocytes and is a subcellular organelle surrounded by a single membrane; >50 peroxisomal enzymes are identified. Some enzymes are involved in the production and decomposition of hydrogen peroxide; others are concerned with lipid and amino acid metabolism. Most peroxisomal enzymes are first synthesized in their mature form on free polyribosomes and enter the cytoplasm. Proteins that are destined for the peroxisome contain specific peroxisome targeting sequences (PTS). Most peroxisomal matrix proteins contain PTS1, a 3-amino acid sequence at the carboxyl terminus. PTS2 is an amino-terminal sequence that is critical for the import of enzymes involved in plasmalogen and branched-chain fatty acid metabolism. Import of proteins involves a complex series of reactions that involves at least 23 distinct proteins. These proteins are referred to as peroxins encoded by PEX genes. Table 80-3 summarizes the PEX genes that are defective in human disease states.
Epidemiology
Except for X-linked adrenoleukodystrophy (X-ALD), all the peroxisomal disorders in Table 80-2 are autosomal recessive traits. X-ALD is the most common peroxisomal disorder, with an estimated incidence of 1/17,000. The combined incidence of the other peroxisomal disorders is estimated to be 1/50,000.
Pathology
Absence or reduction in the number of peroxisomes is pathognomonic for disorders of peroxisome biogenesis. In most disorders, there are membranous sacs that contain peroxisomal integral membrane proteins, which lack the normal complement of matrix proteins; these are peroxisome “ghosts.” Pathologic changes are observed in many organs and include profound and characteristic defects in neuronal migration; micronodular cirrhosis of the liver; renal cysts; chondrodysplasia punctata; corneal clouding, congenital cataracts, glaucoma, and retinopathy; congenital heart disease; and dysmorphic features.
Pathogenesis
It is likely that all pathologic changes are secondary to the peroxisome defect. Multiple peroxisomal enzymes fail to function in the PBD (Table 80-4). The enzymes that are diminished or absent are synthesized but are degraded abnormally fast because they may be unprotected outside of the peroxisome. It is not clear how defective peroxisome functions lead to the widespread pathologic manifestations.
Table 80-4 ABNORMAL LABORATORY FINDINGS COMMON TO DISORDERS OF PEROXISOME BIOGENESIS
Peroxisomes absent to reduced in number
Catalase in cytosol
Deficient synthesis and reduced tissue levels of plasmalogens
Defective oxidation and abnormal accumulation of very long chain fatty acids
Deficient oxidation and age-dependent accumulation of phytanic acid
Defects in certain steps of bile acid formation and accumulation of bile acid intermediates
Defects in oxidation and accumulation of L-pipecolic acid
Increased urinary excretion of dicarboxylic acids
The PBD are associated with genetically determined import defects. The PBD have been subdivided into 12 complementation groups. The molecular defects have been defined in 10 of these groups (see Table 80-3). The pattern and severity of pathologic features vary with the nature of the import defects and the degree to which import is impaired. These gene defects lead to disorders that were named before their relationship to the peroxisome was recognized, namely, Zellweger syndrome (ZS), neonatal adrenoleukodystrophy (NALD), infantile Refsum disease (IRD), and rhizomelic chondrodysplasia punctata (RCDP). The first 3 disorders are considered to form a clinical continuum, with ZS the most severe, IRD the least severe, and NALD intermediate. They can be caused by 11 different gene defects, which involve mainly the import of proteins that contain the PTS1 targeting signal; the gene defects cannot be distinguished on the basis of clinical features. The clinical severity varies with the degree to which protein import is impaired. Mutations that abolish import completely are often associated with the ZS phenotype, whereas a missense mutation, in which some degree of import function is retained, leads to the somewhat milder phenotypes. A defect in PEX7, which involves the import of proteins that utilize PTS2, is associated with RCDP. PEX7 defects that leave import partially intact are associated with milder phenotypes, some of which resemble classic Refsum disease.
PBD With Milder or Atypical Phenotypes
Newborn infants with Zellweger syndrome show striking and consistent, recognizable abnormalities. Of central diagnostic importance are the typical facial appearance (high forehead, unslanting palpebral fissures, hypoplastic supraorbital ridges, and epicanthal folds; Fig. 80-3), severe weakness and hypotonia, neonatal seizures, and eye abnormalities (cataracts, glaucoma, corneal clouding, Brushfield spots, pigmentary retinopathy, and nerve dysplasia). Because of the hypotonia and “mongoloid” appearance, Down syndrome may be suspected. Infants with Zellweger syndrome rarely live more than a few months. More than 90% show postnatal growth failure. Table 80-5 lists the main clinical abnormalities.
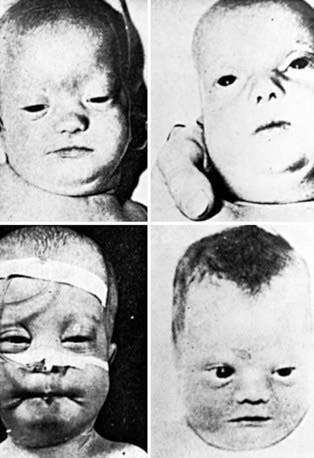
Figure 80-3 Four patients with Zellweger cerebrohepatorenal syndrome. Note the high forehead, epicanthal folds, and hypoplasia of supraorbital ridges and midface.
(Courtesy of Hans Zellweger, MD.)
Patients with neonatal ALD show fewer and, occasionally, no dysmorphic features. Neonatal seizures occur frequently. Some degree of psychomotor development is present; function remains in the severely or profoundly retarded range, and development may regress after 3-5 yr of age, probably from a progressive leukodystrophy. Several patients are now in a stable, albeit disabled, state in their 3rd or 4th decade. Hepatomegaly, impaired liver function, pigmentary degeneration of the retina, and severely impaired hearing are invariably present. Adrenocortical function is usually impaired, but overt Addison disease is rare. Chondrodysplasia punctata and renal cysts are absent.
Patients with infantile Refsum disease have survived to the 2nd decade or longer. They are able to walk, although gait may be ataxic and broad based. Cognitive function is in the severely retarded range. All have sensorineural hearing loss and pigmentary degeneration of the retina. They have moderately dysmorphic features that may include epicanthal folds, a flat bridge of the nose, and low-set ears. Early hypotonia and hepatomegaly with impaired function are common. Levels of plasma cholesterol and high- and low-density lipoprotein are often moderately reduced. Chondrodysplasia punctata and renal cortical cysts are absent. Postmortem study in infantile Refsum disease reveals micronodular liver cirrhosis and small hypoplastic adrenals. The brain shows no malformations, except for severe hypoplasia of the cerebellar granule layer and ectopic locations of the Purkinje cells in the molecular layer. The mode of inheritance is autosomal recessive.
Some patients with PBD disorders have milder and atypical phenotypes. They may present with peripheral neuropathy or with retinopathy, impaired vision, or cataracts in childhood, adolescence, or adulthood and have been diagnosed to have Charcot-Marie-Tooth disease or Usher syndrome. Some patients have survived to the 5th decade. Defects in PEX7, which most commonly lead to the RCDP phenotype, may also lead to a milder phenotype with clinical manifestations similar to those of classical Refsum disease (phytanoyl CoA hydroxylase deficiency).
Rhizomelic Chondrodysplasia Punctata (Rcdp)
This disorder is characterized by the presence of stippled foci of calcification within the hyaline cartilage and is associated with dwarfing, cataracts (72%), and multiple malformations due to contractures. Vertebral bodies have a coronal cleft filled by cartilage that is a result of an embryonic arrest. Disproportionate short stature affects the proximal parts of the extremities (Fig. 80-4A). Radiologic abnormalities consist of shortening of the proximal limb bones, metaphyseal cupping, and disturbed ossification (Fig. 80-4B). Height, weight, and head circumference are less than the 3rd percentile, and these children are severely retarded mentally. Skin changes such as those observed in ichthyosiform erythroderma are present in about 25% of patients.
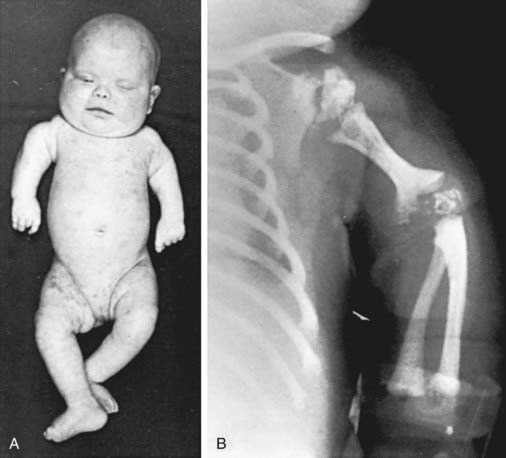
Figure 80-4 A, Newborn infant with rhizomelic chondrodysplasia punctata (RCDP). Note the severe shortening of the proximal limbs, the depressed bridge of the nose, hypertelorism, and widespread scaling skin lesions. B, Note the marked shortening of the humerus and epiphyseal stippling at the shoulder and elbow joints.
(Courtesy of John P. Dorst, MD.)
Isolated Defects of Peroxisomal Fatty Acid Oxidation
The disorders labeled B1 through B3 (see Table 80-2) each involve 1 of 3 enzymes involved in peroxisomal fatty acid oxidation. Their clinical manifestations resemble those of the Zellweger syndrome/neonatal ALD/infantile Refsum disease continuum; they can be distinguished from disorders of peroxisome biogenesis by laboratory tests. Defects of bifunctional enzyme are common and are found in about 15% of patients with the Zellweger syndrome/neonatal ALD/infantile Refsum disease phenotype. Patients with isolated acyl CoA oxidase deficiency have a somewhat milder phenotype that resembles that of neonatal ALD.
Isolated Defects of Plasmalogen Synthesis
Plasmalogens are lipids in which the 1st carbon of glycerol is linked to an alcohol rather than a fatty acid. They are synthesized through a complex series of reactions, the 1st two steps of which are catalyzed by the peroxisomal enzymes dihydroxyacetone phosphate alkyl transferase and synthase. Deficiency of either of these enzymes (B4 and B5 in Table 80-2) leads to a phenotype that is clinically indistinguishable from the peroxisomal import disorder RCDP. This latter disorder is caused by a defect in PEX7, the receptor for peroxisome targeting sequence 2. It shares the severe deficiency of plasmalogens with disorders B4 and B5 but, in addition, has defects of phytanic oxidation. The fact that disorders B4 and B5 are associated with the full phenotype of RCDP suggests that a deficiency of plasmalogens is sufficient to produce it.
Classic Refsum Disease
The defective enzyme (phytanoyl CoA oxidase) is localized to the peroxisome. The manifestation of classic Refsum disease includes impaired vision from retinitis pigmentosa, ichthyosis, peripheral neuropathy, ataxia, and, occasionally, cardiac arrhythmias. In contrast to infantile Refsum disease, cognitive function is normal and there are no congenital malformations. Classic Refsum disease often does not manifest until young adulthood, but visual disturbances such as night blindness, ichthyosis, and peripheral neuropathy may already be present in childhood and adolescence. Early diagnosis is important because institution of a phytanic acid-restricted diet can reverse the peripheral neuropathy and prevent the progression of the visual and central nervous system manifestations. The classical Refsum disease phenotype may also be caused by defects in PEX7.
2-Methylacyl Coa Racemase Deficiency
This disorder is caused by an enzyme defect that leads to the accumulation of the branched-chain fatty acids (phytanic and pristanic acid) and bile acids. Patients present with adult-type peripheral neuropathy and may also have pigmentary degeneration of the retina.
Laboratory Findings
Laboratory tests for peroxisomal disorders can be viewed at three levels of complexity.
Level 1: Does the Patient have a Peroxisomal Disorder?
This can be resolved by noninvasive tests that are generally available (Table 80-6). Measurement of plasma VLCFA is the most commonly used assay. Whereas plasma VLCFA levels are elevated in many patients with peroxisomal disorders, this is not always the case. The most important exceptions are RCDP, in which VLCFA levels are normal, but plasma phytanic acid levels are increased and red blood cell plasmalogen levels are reduced. In some other peroxisomal disorders, the biochemical abnormalities are still more restricted. Therefore, a panel of tests is recommended and includes plasma levels of VLCFA and phytanic, pristanic, and pipecolic acids and red blood cell levels of plasmalogens. Tandem mass spectrometry techniques also permit convenient quantitation of bile acids in plasma and urine. This panel of tests can be performed on 2 mL samples of venous blood and permits detection of most peroxisomal disorders. Furthermore, normal results make the presence of a peroxisomal disorder unlikely.
Level 2: What is the Precise Nature of the Peroxisomal Disorder?
Table 80-6 lists the main biochemical abnormalities in the various peroxisomal disorders. When combined with the clinical presentation, the panel of level 1 tests (see earlier) is often sufficient to identify the precise nature of the defect. Elevated plasma VLCFA levels permit the precise diagnosis of X-ALD in male patients. Marked reduction of erythrocyte plasmalogen levels combined with elevated plasma phytanic acid permits precise diagnosis in a patient with the clinical features of RCDP. Classic Refsum disease can be diagnosed by demonstration of increased plasma phytanic acid combined with normal or reduced levels of pristanic acid levels, while in D-bifunctional enzyme deficiency and 2-methylacyl CoA racemase deficiency, the levels of pristanic and phytanic acid are both increased. Precise identification of some peroxisomal disorders may require more extensive studies in cultured skin fibroblasts. This may be required for the differentiation of PBD from defects in bifunctional enzyme. In PBD, the patient’s peroxisomes are absent and catalase is in the soluble fraction, whereas in bifunctional enzyme defect, peroxisomes are present and catalase is in the particulate fraction. Fibroblast studies are required to identify the nature of the molecular defect in PBD. Whether such specialized studies are clinically warranted depends on individual circumstances. Precise definition of the defect in a proband may improve the precision of prenatal diagnosis in at-risk pregnancies, and it is required for carrier detection. It is also of value in setting prognosis. Precise characterization is of prognostic value in patients with PEX1 defects. This defect is present in approximately 60% of PBD patients, and about half of the PEX1 defects have the G843D allele, which is associated with a significantly milder phenotype than is found in other mutations.
Level 3: What is the Molecular Defect?
Table 80-3 shows that the molecular defects in most of the PBD have been defined. Definition of the molecular defect in the proband, which is now offered in several laboratories, is essential for carrier detection and speeds prenatal diagnosis.
Diagnosis
There are several noninvasive laboratory tests that permit precise and early diagnosis of peroxisomal disorders (see Table 80-6). The challenge in PBD is to differentiate them from the large variety of other conditions that can cause hypotonia, seizures, failure to thrive, or dysmorphic features. Experienced clinicians can readily recognize classic Zellweger syndrome by its clinical manifestations. PBD patients often do not show the full clinical spectrum of disease and may be identifiable only by laboratory assays. Clinical features that may serve as indications for these diagnostic assays include severe psychomotor retardation; weakness and hypotonia; dysmorphic features; neonatal seizures; retinopathy, glaucoma, or cataracts; hearing deficits; enlarged liver and impaired liver function; and chondrodysplasia punctata. The presence of one or more of these abnormalities increases the likelihood of this diagnosis. Atypical milder forms presenting as peripheral neuropathy have also been described.
Patients with RCDP must be distinguished from patients with other causes of chondrodysplasia punctata. In addition to warfarin embryopathy and Zellweger syndrome, these disorders include the milder autosomal dominant form of chondrodysplasia punctata (Conradi-Hünermann syndrome), which is characterized by longer survival, absence of severe limb shortening, and usually intact intellect; an X-linked dominant form; and an X-linked recessive form associated with a deletion of the terminal portion of the short arm of the X chromosome. RCDP is suspected clinically because of the shortness of limbs, psychomotor retardation, and ichthyosis. The most decisive laboratory test is the demonstration of abnormally low plasmalogen levels in red blood cells and an impaired capacity to synthesize plasmalogens in cultured skin fibroblasts. These biochemical defects are not present in other types of chondrodysplasia punctata. Chondrodysplasia punctata may also be associated with a defect of 3β-hydroxysteroid-Δ8,Δ7-isomerase, an enzyme involved in biosynthesis of cholesterol.
Complications
Patients with Zellweger cerebrohepatorenal syndrome have multiple disabilities involving muscle tone, swallowing, cardiac abnormalities, liver disease, and seizures. These conditions are treated symptomatically, but the prognosis is poor, and most patients succumb in the 1st few months of life. Patients with RCDP may develop quadriparesis owing to compression at the base of the brain.
Treatment
The most effective therapy is the dietary treatment of classic Refsum disease with a phytanic acid-restricted diet.
For patients with the somewhat milder variants of the peroxisome import disorders, success has been achieved with multidisciplinary early intervention, including physical and occupational therapy, hearing aids, alternative communication, nutrition, and support for the parents. Although most patients continue to function in the profoundly or severely retarded range, some make significant gains in self-help skills, and several are in stable condition in their teens or even early 20s.
Studies to mitigate some of the secondary biochemical abnormalities include the oral administration of docosahexaenoic acid in a dosage of 50-100 mg/24 hr either as the ethyl ester or in the form of a triglyceride in which one of the fatty acids has been replaced by docosahexaenoic acid. The levels of this substance are greatly reduced in patients with disorders of peroxisome biogenesis because the last step of its synthesis takes place in the peroxisome. This therapy normalizes the plasma and erythrocyte levels of this substance, which has important physiologic functions in retina and brain. There are anecdotal reports of clinical improvement. The oral administration of cholic acid and chenodeoxycholic acid in a dosage of 100-250 mg/24 hr, with the aim of reducing the levels of presumably toxic bile acid intermediates, may be effective as well.
Genetic Counseling
All the peroxisomal disorders, except hyperoxaluria type 1, can be diagnosed prenatally in the 1st or 2nd trimester. The tests are similar to those described for postnatal diagnosis (see Table 80-6) and use chorionic villus sampling or amniocytes. More than 300 pregnancies have been monitored, and more than 60 affected fetuses have been identified without diagnostic error. Because of the 25% recurrence risk, couples with an affected child must be advised about the availability of prenatal diagnosis. Heterozygotes can be identified in X-ALD and in those disorders in which the molecular defect has been identified (see Table 80-3).
Adrenoleukodystrophy (X-Linked)
X-ALD is a genetically determined disorder associated with the accumulation of saturated VLCFA and a progressive dysfunction of the adrenal cortex and central and peripheral nervous system white matter.
Etiology
The key biochemical abnormality is the tissue accumulation of unbranched saturated VLCFA, with a carbon chain length of 24 or more. Excess hexacosanoic acid (C26 : 0) is the most striking and characteristic feature. This accumulation of fatty acids is caused by genetically deficient peroxisomal degradation of fatty acid. The key biochemical defect involves the impaired function of peroxisomal lignoceroyl CoA ligase, the enzyme that catalyzes the formation of the CoA derivative of VLCFA. The gene that is defective (ABCD1) codes for a peroxisomal membrane (ALDP). More than 400 distinct mutations have been identified, and most families have a mutation that is “private” (unique to that kindred) and are updated on the website, www.x-ald.nl. The gene has been mapped to chromosome Xq28. The mechanism by which the ALDP defect leads to VLCFA accumulation and the pathology of X-ALD is unknown.
Epidemiology
The minimum incidence of X-ALD in males is 1/21,000, and the combined incidence of X-ALD males and heterozygous females in the general population is estimated to be 1/17,000. All races are affected. The various phenotypes often occur in members of the same kindred.
Pathology
Characteristic lamellar cytoplasmic inclusions can be demonstrated with the electron microscope in adrenocortical cells, testicular Leydig cells, and nervous system macrophages. These inclusions probably consist of cholesterol esterified with VLCFA. They are most prominent in cells of the zona fasciculata of the adrenal cortex, which at first are distended with lipid and later atrophy.
The nervous system can display 2 types of lesions. In the severe childhood cerebral form and in the rapidly progressive adult forms, demyelination is associated with an inflammatory response manifested by the accumulation of perivascular lymphocytes that is most intense in the parieto-occipital region. In the slowly progressive adult form, adrenomyeloneuropathy (AMN), the main finding is a distal axonopathy that affects the long tracts in the spinal cord. The inflammatory response is mild or absent.
Pathogenesis
The adrenal dysfunction is probably a direct consequence of the accumulation of VLCFA. The cells in the zona fasciculata are distended with abnormal lipids. Cholesterol esterified with VLCFA is relatively resistant to adrenocorticotropic hormone (ACTH)-stimulated cholesterol ester hydrolases, and this limits the capacity to convert cholesterol to active steroids. In addition, C26 : 0 excess increases the viscosity of the plasma membrane and this may interfere with receptor and other cellular functions.
There is no correlation between the neurologic phenotype and the nature of the mutation or the severity of the biochemical defect as assessed by plasma levels of VLCFA or between the degree of adrenal involvement and nervous system involvement. The severity of the illness and rate of progression correlate with the intensity of the inflammatory response. The inflammatory response may be cytokine mediated and may involve an autoimmune response triggered in an unknown way by the excess of VLCFA. A CD1 lipid antigen has been implicated. Mitochondrial damage and oxidative stress also contribute. Approximately half of the patients do not experience the inflammatory response. A modifier gene that sets the “thermostat” for the inflammatory response is postulated.
Clinical Manifestations
There are 5 relatively distinct phenotypes, 3 of which are present in childhood with symptoms and signs. In all the phenotypes, development is usually normal in the 1st 3-4 yr of life.
In the childhood cerebral form of ALD, symptoms are first noted most commonly between the ages of 4 and 8 yr (21 mo is the earliest onset reported). The most common initial manifestations are hyperactivity, which is often mistaken for an attention deficit disorder, and worsening school performance in a child who had previously been a good student. Auditory discrimination is often impaired, although tone perception is preserved. This may be evidenced by difficulty in using the telephone and greatly impaired performance on intelligence tests in items that are presented verbally. Spatial orientation is often impaired. Other initial symptoms are disturbances of vision, ataxia, poor handwriting, seizures, and strabismus. Visual disturbances are often due to involvement of the cerebral cortex, which leads to variable and seemingly inconsistent visual capacity. Seizures occur in nearly all patients and may represent the 1st manifestation of the disease. Some patients present with increased intracranial pressure or with unilateral mass lesions. Impaired cortisol response to ACTH stimulation is present in 85% of patients, and mild hyperpigmentation is noted. In most patients with this phenotype, adrenal dysfunction is recognized only after the condition is diagnosed because of the cerebral symptoms. Cerebral childhood ALD tends to progress rapidly with increasing spasticity and paralysis, visual and hearing loss, and loss of ability to speak or swallow. The mean interval between the 1st neurologic symptom and an apparently vegetative state is 1.9 yr. Patients may continue in this apparently vegetative state for 10 yr or more.
Adolescent ALD designates patients who experience neurologic symptoms between the ages of 10 and 21 yr. The manifestations resemble those of childhood cerebral ALD except that progression is slower. About 10% of patients present acutely with status epilepticus, adrenal crisis, acute encephalopathy, or coma.
Adrenomyeloneuropathy first manifests in late adolescence or adulthood as a progressive paraparesis caused by long tract degeneration in the spinal cord. Approximately half of the patients also have involvement of the cerebral white matter.
The “Addison only” phenotype is an important and underdiagnosed condition. Of male patients with Addison disease, 25% may have the biochemical defect of ALD. Many of these patients have intact neurologic systems, whereas others have subtle neurologic signs. Many acquire adrenomyeloneuropathy in adulthood.
The term “asymptomatic ALD” is applied to persons who have the biochemical defect of ALD but are free of neurologic or endocrine disturbances. Nearly all persons with the gene defect eventually become neurologically symptomatic. A few have remained asymptomatic even in the 6th or 7th decade.
Approximately 50% of female heterozygotes acquire a syndrome that resembles adrenomyeloneuropathy but is milder and of later onset. Adrenal insufficiency is rare.
Laboratory and Radiographic Findings
The most specific and important laboratory finding is the demonstration of abnormally high levels of VLCFA in plasma, red blood cells, or cultured skin fibroblasts. The test should be performed in a laboratory that has experience with this specialized procedure. Positive results are obtained in all male patients with X-ALD and in about 85% of female carriers of X-ALD. Mutation analysis is the most reliable method for the identification of carriers.
CT And MRI
Patients with childhood cerebral or adolescent ALD show cerebral white matter lesions that are characteristic with respect to location and attenuation patterns on MRI. In 80% of patients, the lesions are symmetric and involve the periventricular white matter in the posterior parietal and occipital lobes. About 50% show location of a garland of accumulated contrast material adjacent and anterior to the posterior hypodense lesions (Fig. 80-5A). This zone corresponds to the zones of intense perivascular lymphocytic infiltration where the blood-brain barrier breaks down. In 12% of patients, the initial lesions are frontal. Unilateral lesions that produce a mass effect suggestive of a brain tumor may occur. MRI provides a clearer delineation of normal and abnormal white matter than does CT and may demonstrate abnormalities missed by CT (Fig. 80-5B).
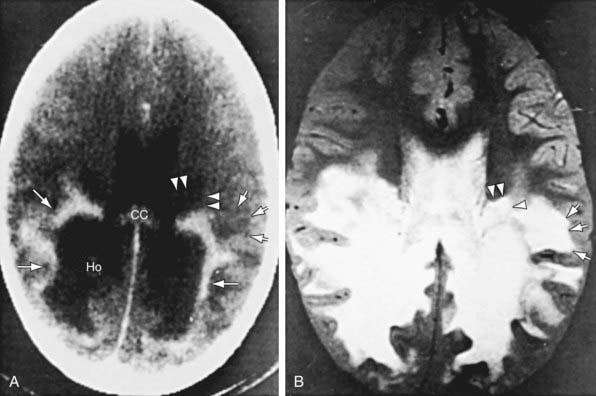
Figure 80-5 A, Contrast enhanced CT abnormalities in adrenoleukodystrophy (ALD) with typical parieto-occipital location, showing symmetric bilateral hypodense inactive zones (Ho). The enhancing active periphery zone of hypodensity is demarcated by arrows. Compare the anterior zone of hypodensity (arrowheads) with that on the MRI in B. CC, corpus callosum. B, MRI of the same pattern and area shown by CT. MRI T2 weighted image shows a high-intensity signal of the abnormally bright parieto-occipital white matter. Subcortical involvement is better identified on MRI. Separation of active zones may be better appreciated by CT, because both inactive and active zones are seen at high-signal areas on MRI. It is assumed, however, that such major distinctions afforded by CT will also be demonstrable when IV enhancement (paramagnetic enhancement) becomes readily available. Note the hypodense involvement of CT (arrowheads and arrows in A) compared with the well-resolved lesions on MRI in B.
(From Kumar AJ, Rosenbaum WE, Naidu S, et al: Adrenoleukodystrophy: corresponding MR imaging with CT, Radiology 165:497–504, 1987.)
Diagnosis and Differential Diagnosis
The earliest manifestations of childhood cerebral ALD are difficult to distinguish from the more common attention-deficit disorders or learning disabilities. Rapid progression, signs of dementia, or difficulty in auditory discrimination suggest ALD. Even in early stages, CT or MRI may show strikingly abnormal changes. Other leukodystrophies (Chapters 592 and 605.10) or multiple sclerosis (Chapter 593.1) may mimic these radiographic findings. Definitive diagnosis depends on demonstration of VLCFA excess, which occurs only in X-ALD and the other peroxisomal disorders. The latter may be distinguished from X-ALD by their clinical presentation during the neonatal period.
Adolescent or adult cerebral ALD can be confused with psychiatric disorders, dementing disorders, or epilepsy. The 1st clue to the diagnosis of ALD may be the demonstration of white matter lesions by CT or MRI; assays of VLCFA are confirmatory.
ALD cannot be distinguished clinically from other forms of Addison disease; it is recommended that assays of VLCFA levels be performed in all male patients with Addison disease. ALD patients do not usually have antibodies to adrenal tissue in their plasma.
Complications
An avoidable complication is the occurrence of adrenal insufficiency. The most difficult neurologic problems are those related to bed rest, contracture, coma, and swallowing disturbances. Other complications involve behavioral disturbances and injuries associated with defects of spatial orientation, impaired vision and hearing, and seizures.
Treatment
Corticosteroid replacement for adrenal insufficiency or adrenocortical hypofunction is effective (Chapter 569). It may be lifesaving and increase general strength and well-being, but it does not alter the course of the neurologic disability.
Bone Marrow Transplantation
Bone marrow transplantation (BMT) benefits patients who show early evidence of the inflammatory demyelination that is characteristic of the rapidly progressive neurologic disability in boys and adolescents with the cerebral X-ALD phenotype. BMT is a high-risk procedure, and patients must be selected with great care. The mechanism of the beneficial effect is incompletely understood. Bone marrow-derived cells do express ALDP, the protein that is deficient in X-ALD; approximately 50% of brain microglial cells are bone marrow derived. It is possible that replacement of affected cells by cells that contain the normal gene changes the brain milieu sufficiently to correct the brain metabolic disturbance. The favorable effect may also be caused by modification of the brain inflammatory response. Five to 10 yr follow-up of boys and adolescents who had early cerebral involvement has shown stabilization and, in some instances, improvement. On the other hand, BMT has not shown favorable effects in patients who had already severe brain involvement and may accelerate disease progression under these circumstances. The nonverbal IQ has been found to be of predictive value, and transplant is not recommended in patients with nonverbal IQ significantly below 80. Unfortunately, in more than half the patients who are diagnosed because of neurologic symptoms, the illness is so advanced that they are not candidates for transplant.
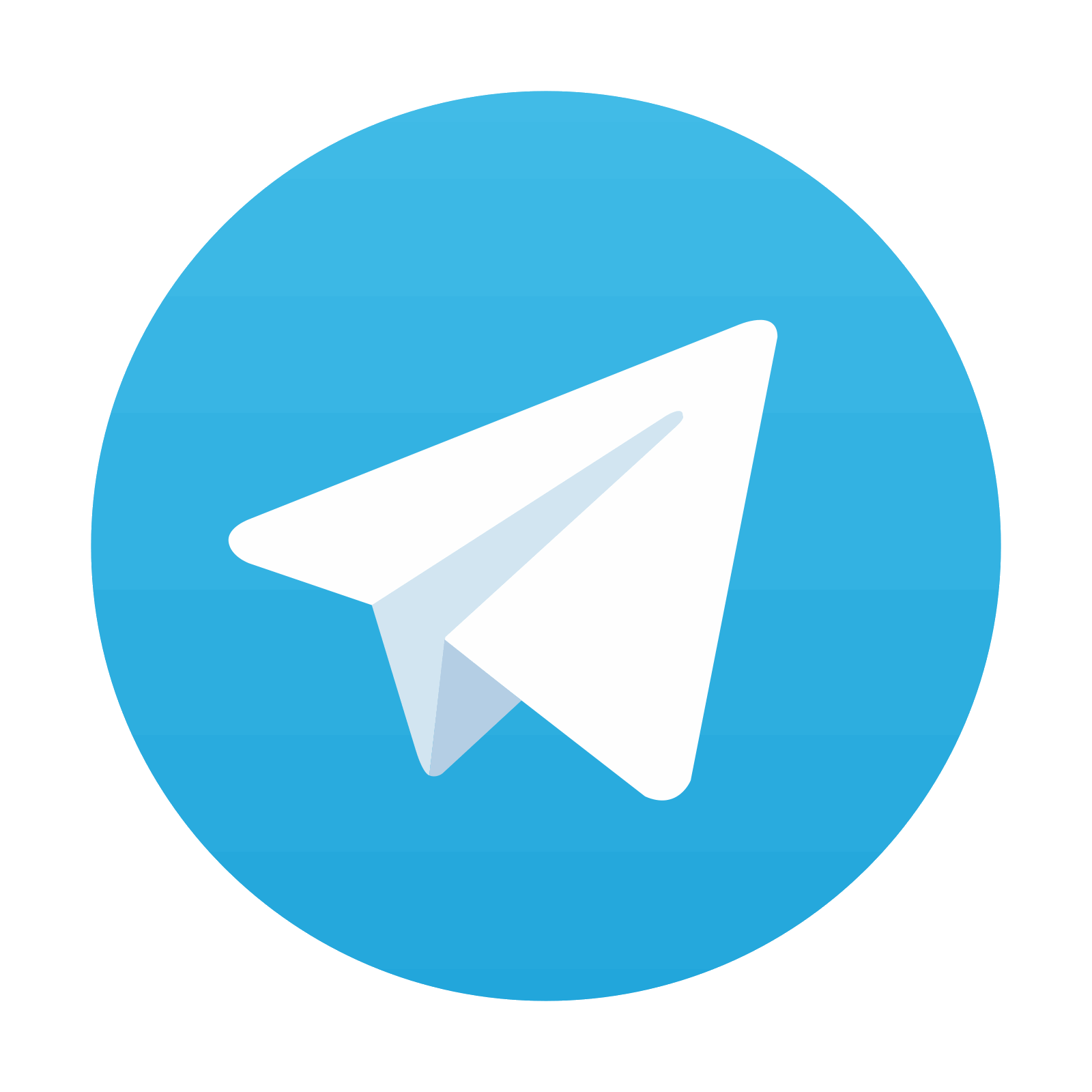
Stay updated, free articles. Join our Telegram channel
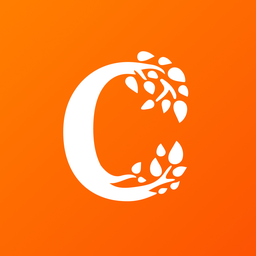
Full access? Get Clinical Tree
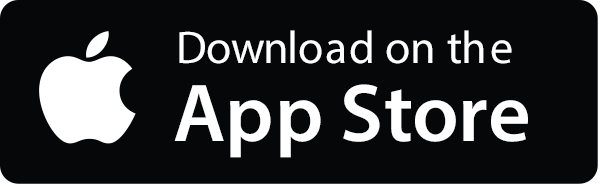
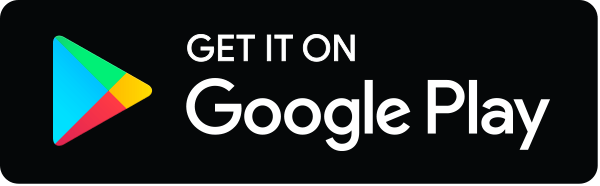