Chapter 79 Defects in Metabolism of Amino Acids
79.1 Phenylalanine
Phenylalanine is an essential amino acid. Dietary phenylalanine not utilized for protein synthesis is normally degraded by way of the tyrosine pathway (Fig. 79-1). Deficiency of the enzyme phenylalanine hydroxylase (PAH) or of its cofactor tetrahydrobiopterin (BH4) causes accumulation of phenylalanine in body fluids and in the brain. The severity of hyperphenylalaninemia depends on the degree of enzyme deficiency and may vary from very high plasma concentrations (>20 mg/dL or >1,200 µmole/L, classic phenylketonuria [PKU]) to mildly elevated levels (2-6 mg/dL or 120-360 µmole/L). In affected infants with plasma concentrations >20 mg/dL, excess phenylalanine is metabolized to phenylketones (phenylpyruvate and phenylacetate; see Fig. 79-1) that are excreted in the urine, giving rise to the term phenylketonuria (PKU). These metabolites have no role in pathogenesis of central nervous system (CNS) damage in patients with PKU; their presence in the body fluids simply signifies the severity of the condition. The term hyperphenylalaninemia implies lower plasma levels (<20 mg/dL) of phenylalanine; these patients may or may not need dietary therapy based on their blood phenylalanine level. The brain is the main organ affected by hyperphenylalaninemia. The CNS damage in affected patients is caused by the elevated concentration of phenylalanine in brain tissue. The high blood levels of phenylalanine in PKU saturate the transport system across the blood-brain barrier causing inhibition of the cerebral uptake of other large neutral amino acids such as tyrosine and tryptophan. The exact mechanism of damage caused by elevated levels of intracerebral phenylalanine remains elusive. There are a few adults with classic PKU and normal intelligence who have never been treated with a phenylalanine-restricted diet. Phenylalanine content of the brain in these individuals was found to be close to that of normal subjects when studied by magnetic resonance spectroscopy (MRS) and imaging (MRI) techniques.

Figure 79-1 Pathways of phenylalanine and tyrosine metabolism. Enzyme defects causing genetic conditions are depicted as horizontal bars crossing the reaction arrow(s). Pathways for synthesis of cofactor BH4 are shown in purple. PKU* refers to defects of BH4 metabolism that affect the phenylalanine, tyrosine, and tryptophan hydroxylases (see Figs. 79-2 and 79-5). Enzymes: (1) phenylalanine hydroxylase (PAH), (2) pterin-carbinolamine dehydratase (PCD), (3) dihydrobiopterin reductase, (4) guanosine triphosphate (GTP) cyclohydrolase, (5) 6-pyruvoyltetrahydropterin synthase (6-PTS), (6) seriapterin reductase, (7) carbonyl reductase, (8) aldolase reductase, (9) dihydrofolate reductase, (10) tyrosine aminotransferase, (11a) intramolecular rearrangement, (11) 4-hydroxyphenylpyruvate dioxygenase, (12) homogentisic acid dioxygenase, (13) maleylacetoacetate isomerase, (14) fumarylacetoacetate hydroxylase, (NE) nonenzymatic.
Classic Phenylketonuria
Severe hyperphenylalaninemia (plasma phenylalanine levels >20 mg/dL), if untreated, invariably results in the development of signs and symptoms of classic PKU, except in rare unpredictable cases.
Clinical Manifestations
The affected infant is normal at birth. Profound mental retardation develops gradually if the infant remains untreated. Cognitive delay may not be evident for the 1st few months. In untreated patients, 50-70% will have an IQ below 35, and 88-90% below 65. Only 2-5% of untreated patients will have normal intelligence. Many patients require institutional care if the condition remains untreated. Vomiting, sometimes severe enough to be misdiagnosed as pyloric stenosis, may be an early symptom. Older untreated children become hyperactive with autistic behaviors, including purposeless hand movements, rhythmic rocking, and athetosis.
The infants are lighter in their complexion than unaffected siblings. Some may have a seborrheic or eczematoid rash, which is usually mild and disappears as the child grows older. These children have an unpleasant odor of phenylacetic acid, which has been described as musty or mousey. Neurologic signs include seizures (≈25%), spasticity, hyperreflexia, and tremors; more than 50% have electroencephalographic abnormalities. Microcephaly, prominent maxillae with widely spaced teeth, enamel hypoplasia, and growth retardation are other common findings in untreated children. The clinical manifestations of classic PKU are rarely seen in those countries in which neonatal screening programs for the detection of PKU are in effect.
Milder Forms of Hyperphenylalaninemia, Non-PKU Hyperphenylalaninemias
In any screening program for PKU, a group of infants are identified in whom initial plasma concentrations of phenylalanine are above normal (2 mg/dL, 120 µmole/L) but <20 mg/dL (1,200 µmole/L). These infants do not excrete phenylketones. The term hyperphenylalaninemia implies lower plasma concentration of phenylalanine, but these patients may still require dietary therapy depending on their untreated plasma phenylalanine level. Attempts have been made to classify these patients in different subgroups depending on the degree of hyperphenylalaninemia, but such a practice has little clinical or therapeutic advantage. The possibility of deficiency of BH4 should be investigated in all infants with the milder forms of hyperphenylalaninemia (see later).
Diagnosis
Because of the often gradual development of clinical manifestations, hyperphenylalaninemia is usually diagnosed through mass screening of newborn infants. In infants with positive results from the screen for hyperphenylalaninemia, diagnosis should be confirmed by quantitative measurement of plasma phenylalanine concentration. Identification and measurement of phenylketones in the urine has no place in any screening program. In countries and places where such programs are not in effect, identification of phenylketones in the urine by ferric chloride may offer a simple test for diagnosis of infants with developmental and neurologic abnormalities. Once the diagnosis of hyperphenylalaninemia is established, additional studies for biopterin metabolism should be performed to rule out biopterin deficiency as the cause of hyperphenylalaninemia (see later).
Neonatal Screening for Hyperphenylalaninemia
Effective and relatively inexpensive methods for mass screening of newborn infants have been developed and are used in the USA and several other countries. A few drops of blood, which are placed on a filter paper and mailed to a central laboratory, are used for assay. The bacterial inhibition assay of Guthrie, which was the 1st method used for this purpose, has been replaced by more precise and quantitative methods (fluorometric and tandem mass spectrometry). The method of choice is tandem mass spectrometry (MS/MS), which identifies all forms of hyperphenyalaninemia with a low false-positive rate, and excellent accuracy and precision. The addition of the phenylalanine/tyrosine molar ratio has further reduced the number of false-positive results. Diagnosis must be confirmed by measurement of plasma phenylalanine concentration. Blood phenylalanine in affected infants with PKU may rise to diagnostic levels as early as 4 hr after birth even in the absence of protein feeding. It is recommended that the blood for screening be obtained in the 1st 24-48 hr of life after feeding protein to reduce the possibility of false-negative results, especially in the milder forms of the condition.
Treatment
The goal of therapy is to reduce phenylalanine levels in the plasma and brain. It is generally accepted that infants with persistent (more than a few days) plasma levels of phenylalanine >6 mg/dL (360 µmole/L) should be treated with a phenylalanine-restricted diet similar to that for classic PKU. Formulas low in or free of phenylalanine are commercially available. The diet should be started as soon as diagnosis is established. Because phenylalanine is not synthesized endogenously, small amounts of phenylalanine should be added to the diet to prevent phenylalanine deficiency. Dietary deficiency of this amino acid is manifested by lethargy, failure to thrive, anorexia, anemia, rashes, diarrhea, and even death; moreover, tyrosine becomes an essential amino acid in this disorder and its adequate intake must be ensured. Special food items low in phenylalanine is now commercially available for dietary treatment of affected children and adults.
There is no firm consensus concerning optimal level of blood phenylalanine in affected patients either across different countries or among treatment centers in the USA. In 2001, the National Institutes of Health Consensus Development Panel recommended that plasma phenylalanine levels to be maintained between 2 and 6 mg/dL in neonates through 12 yr of age and between 2 and 15 mg/dL in older individuals. The fact that brain development continues in adolescence and even in adulthood, lower plasma phenylalanine levels (2-10 mg/dL) have been encouraged strongly after 12 yr of age. The duration of diet therapy is also controversial. Discontinuation of therapy, even in adulthood, may cause deterioration of IQ and cognitive performance. The current recommendation from the 2001 National Institutes of Health Consensus Development Panel is that all patients be kept on a phenylalanine-restricted diet for life.
Given the difficulty of maintaining a strict low-phenylalanine diet, there are continuing attempts to find other modalities for treatment of these patients. Oral administration of tetrahydrobiopterin (BH4), the cofactor for PAH, may result in reduction of plasma levels of phenylalanine in some patients with PAH deficiency. Plasma levels of phenylalanine in these patients may decrease enough to allow for considerable modification of their dietary restriction. In very rare cases, the diet may be discontinued since the phenylalanine levels remain under 6 mg/dL. The response to BH4 cannot be predicted consistently on the basis of genotype, especially in compound heterozygous patients. Sapropterin, a synthetic form of BH4, which acts as a cofactor in patients with residual PAH activity, is approved by the Food and Drug Administration (FDA) to reduce phenylalanine levels in PKU. At a dose of 10 mg/kg/day, it reduces phenylalanine levels in up to 50% of patients.
Long-term care of these patients is best achieved by a team of experienced professionals (physician specialist, nutritionist, neurologist, geneticist, and psychologist) in a regional treatment center.
Pregnancy in Women With Hyperphenylalaninemia (Maternal PKU)
Pregnant women with hyperphenylalaninemia who are not on a phenylalanine-restricted diet have a very high risk of having offspring with mental retardation, microcephaly, growth retardation, and congenital heart disease. These complications are directly correlated with elevated maternal phenylalanine levels during pregnancy. Prospective mothers who have been treated for hyperphenylalaninemia should be maintained on a phenylalanine-restricted diet before and during pregnancy; every effort should be made to keep blood phenylalanine levels below 6 mg/dL (360 µmole/L) throughout the pregnancy. All women with hyperphenylalaninemia who are of childbearing age should be counseled properly as to the risk of the just described congenital anomalies in their offspring.
Hyperphenylalaninemia due to Deficiency of the Cofactor BH4
In 1-3% of infants with hyperphenylalaninemia, the defect resides in 1 of the enzymes necessary for production or recycling of the cofactor BH4 (Fig. 79-2). If these infants are misdiagnosed as having PKU, they may deteriorate neurologically despite adequate control of plasma phenylalanine. BH4 is synthesized from guanosine triphosphate (GTP) through several enzymatic reactions (see Fig. 79-1). In addition to acting as a cofactor for PAH, BH4 is also a cofactor for tyrosine hydroxylase and tryptophan hydroxylase, which are involved in the biosynthesis of dopamine (see Fig. 79-2) and serotonin (see Fig 79-5), respectively. Therefore, patients with hyperphenylalaninemia due to BH4 deficiency also manifest neurologic findings related to deficiencies of the neurotransmitters dopamine and serotonin. Four enzyme deficiencies leading to defective BH4 formation cause hyperphenylalaninemia and deficiencies of dopamine and serotonin. These include autosomal recessive GTP cyclohydrolase deficiency, pterin-carbinolamine dehydratase (PCD) deficiency, dihydropteridine reductase (DHPR) deficiency, and 6-pyruvoyltetrahydropterin synthase (PTPS or 6-PTS) deficiency. More than half of the reported patients have had a deficiency of 6-pyruvoyltetrahydropterin synthase. Autosomal dominant form of GTP deficiency and sepiapterin reductase deficiency result in deficiencies of neurotransmitters without hyperphenylalaninemia (Chapter 79.11 and Fig. 79-1).
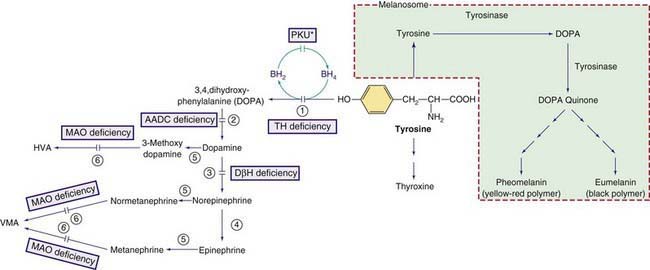
Figure 79-2 Other pathways involving tyrosine metabolism. PKU* indicates hyperphenylalanemia due to tetrahydrobiopterin (BH4) deficiency (see Fig. 79-1). HVA, homovanillic acid; VMA, vanillymandelic acid. Enzymes: (1) tyrosine hydroxylase (TH), (2) aromatic L-amino acid decarboxylase (AADC), (3) dopamine hydroxylase, (4) phenylethanolamine-N-methyltransferase (PNMT), (5) catechol O-methyltransferase (COMT), (6) Monoamine oxidase (MAO).
Clinical Manifestations
Infants with cofactor deficiency are identified during screening programs for PKU because of evidence of hyperphenylalaninemia. Plasma phenylalanine levels may be as high as those in classic PKU or in the range of milder forms of hyperphenylalaninemia. However, the clinical manifestations of the neurotransmitter disorders differ greatly from those of PKU. Neurologic symptoms of the neurotransmitter disorders often manifest in the 1st few months of life and include extrapyramidal signs with choreoathetotic or dystonic limb movements, axial and truncal hypotonia, hypokinesia, feeding difficulties, and autonomic problems. Mental retardation, seizures, hypersalivation, and swallowing difficulties are also seen. The symptoms are usually progressive and often have a marked diurnal fluctuation.
Diagnosis
BH4 deficiency and the responsible enzyme defect may be diagnosed by the following studies:
Treatment
The goals of therapy are to correct hyperphenylalaninemia and to restore neurotransmitter deficiencies in the CNS. The control of hyperphenylalaninemia is important in patients with cofactor deficiency, because high levels of phenylalanine interfere with the transport of neurotransmitter precursors (tyrosine, tryptophan) into the brain. Plasma phenylalanine should be maintained as close to normal as possible (<6 mg/dL). This can be achieved by a combination of a low phenylalanine diet and oral supplementation of BH4. Infants with GTP cyclohydrolase or 6-PTS deficiencies respond more readily to BH4 therapy (5-10 mg/kg/day) than those with dihydropteridine reductase deficiency. In the latter patients, doses as high as 20 mg/kg/day may be required. BH4 for replacement therapy is commercially available, although it is expensive.
Lifelong supplementation with neurotransmitter precursors such as L-dopa and 5-hydroxytryptophan, along with carbidopa to inhibit degradation of L-dopa before it enters the CNS, is necessary in most of these patients even when treatment with BH4 normalizes plasma levels of phenylalanine. BH4 does not readily enter the brain to restore neurotransmitter production. Supplementation with folinic acid is also recommended in patients with dihydropteridine reductase deficiency. Unfortunately, attempting to normalize neurotransmitter levels using neurotransmitter precursors usually does not fully resolve the neurologic symptoms due to the inability to attain normal levels of BH4 in the brain. Patients often demonstrate mental retardation, fluctuating abnormalities of tone, eye movement abnormalities, poor balance and coordination, decreased ability to ambulate, and seizures in spite of supplementation with neurotransmitter precursors.
Hyperprolactinemia occurs in patients with BH4 deficiency and may be due to hypothalamic dopamine deficiency. Measurement of serum prolactin levels may be a convenient method for monitoring adequacy of neurotransmitter replacement in affected patients.
Some drugs such as trimethoprim sulfamethoxazole, methotrexate, and other antileukemic agents are known to inhibit dihydropteridine reductase enzyme activity and should be used with great caution in patients with BH4 deficiency.
Genetics and Prevalence
All defects causing hyperphenylalaninemia are inherited as autosomal recessive traits. The prevalence of PKU in the USA is estimated at 1/14,000 to 1/20,000 live births. The prevalence of non-PKU hyperphenylalaninemia is estimated at 1/50,000. The condition is more common in whites and Native Americans and less prevalent in blacks, Hispanics, and Asians.
The gene for PAH is located on chromosome 12q24.1 and many disease-causing mutations have been identified in different families. The majority of patients are compound heterozygotes for 2 different mutant alleles. The gene for PTP synthase, the most common cause of BH4 deficiency, resides on chromosome 11q22.3-23.3, the gene for dihydropteridine reductase is located on chromosome 4p15.3, and those of carbinolamine dehydratase and GTP cyclohydrolase are on 10q22 and 14q22.1-22.2, respectively. Many disease-causing mutations of these genes have been identified. Prenatal diagnosis is possible using specific genetic probes in cells obtained from chorionic villi biopsy.
Blau N. Defining tetrahydrobiopterin (BH4)-responsiveness in PKU. J Inherit Metab Dis. 2008;31:2-3.
Blau N, Bélanger-Quintana A, Demirkol M, et al. Optimizing the use of sapropterin (BH4) in the management of phenylketonuria. Mol Genet Metab. 2009;96:158-163.
Blau N, Bélanger-Quintana A, Demirkol M, et al. Management of phenylketonuria in Europe: survey results from 19 countries. Mol Genet Metab. 2010;99:109-115.
Blau N, van Spronsen FJ, Levy HL. Phenylketonuria. Lancet. 2010;376:1417-1427.
Burton BK, Adams DJ, Grange DK, et al. Tetrahydrobiopterin therapy for phenylketonuria in infants and young children. J Pediatr. 2011;158:410-415.
Cederbaum S. Tetrahydrobiopterin and PKU: into the future. J Pediatr. 2011;158:351-353.
Committee on Genetics. Maternal phenylketonuria. Pediatrics. 2008;122:445-449.
Feillet F, van Spronsen FJ, MacDonald A, et al. Challenges and pitfalls in the management of phenylketonuria. Pediatrics. 2010;126:333-341.
Giovannini M, Verduci E, Salvatici E, et al. Phenylketonuria: dietary and therapeutic challenges. J Inherit Metab Dis. 2007;30:145-152.
Mitchell JJ, Scriver CR. Phenylalanine hydroxylase deficiency, 2010. GeneReviews at GeneTests: Medical Information Resource. University of Washington, Seattle, 1997-2010. www.genetests.org. Accessed May 2010
Scriver CR, Levy H, Donlon J. Hyperphenylalaninemia: phenylalanine hydroxylase deficiency. In: Scriver CR, Beaudet AL, Sly WS, et al, editors. The metabolic and molecular bases of inherited disease. New York: McGraw-Hill, 2008.
Waisbren SE, Noel K, Fahrbach K, et al. Phenylalanine blood levels and clinical outcomes in phenylketonuria: a systematic literature review and meta-analysis. Mol Genet Metab. 2007;92:63-70.
Waisbren SE, White D, van Spronsen F. Phenylketonuria, psychology and the brain. Mol Genet Metab. 2010;99(Suppl):S1-S108.
Zurfluh MR, Zschocke J, Lindner M, et al. Molecular genetics of tetrahydrobiopterin-responsive phenylalanine hydroxylase deficiency. Hum Mutat. 2008;29:167-175.
79.2 Tyrosine
Tyrosine is derived from ingested proteins or is synthesized endogenously from phenylalanine. It is used for protein synthesis and is a precursor of dopamine, norepinephrine, epinephrine, melanin, and thyroxine. Excess tyrosine is metabolized to carbon dioxide and water (see Fig. 79-1). Hereditary causes of hypertyrosinemia include deficiencies of tyrosine aminotransferase, 4-hydroxyphenylpyruvate dioxygenase (4-HPPD), or fumarylacetoacetate hydrolase (FAH). Acquired hypertyrosinemia may occur in severe hepatocellular dysfunction (liver failure), scurvy (vitamin C is the cofactor for the enzyme 4-HPPD), and hyperthyroidism. Hypertyrosinemia is common in blood samples obtained soon after eating.
Tyrosinemia Type I (Tyrosinosis, Hereditary Tyrosinemia, Hepatorenal Tyrosinemia)
This severe disease of the liver, kidney, and peripheral nerve, is caused by a deficiency of the enzyme FAH. Organ damage is believed to result from accumulation of metabolites of tyrosine degradation, especially succinylacetone.
Clinical Manifestations
Untreated, the affected infant appears normal at birth and typically presents between 2 and 6 mo of age but rarely may become symptomatic in the 1st mo or appear healthy beyond the 1st yr of life. The earlier the presentation, the poorer is the prognosis. The 1 yr mortality, which is about 60% in infants who develop symptoms before 2 mo of age, decreases to 4% in infants who become symptomatic after 6 mo of age.
An acute hepatic crisis commonly heralds the onset of the disease and is usually precipitated by an intercurrent illness that produces a catabolic state. Fever, irritability, vomiting, hemorrhage, hepatomegaly, jaundice, elevated levels of serum transaminases, and hypoglycemia are common. An odor resembling boiled cabbage may be present, due to increased methionine metabolites. Most hepatic crises resolve spontaneously, but may progress to liver failure and death. Between the crises, varying degrees of failure to thrive, hepatomegaly, and coagulation abnormalities often persist. Cirrhosis and eventually hepatocellular carcinoma occur with increasing age. Carcinoma is unusual before 2 yr of age.
Episodes of acute peripheral neuropathy resembling acute porphyria occur in ≈40% of affected children. These crises, often triggered by a minor infection, are characterized by severe pain, often in the legs, associated with hypertonic posturing of the head and trunk, vomiting, paralytic ileus, and, occasionally, self-induced injuries of the tongue or buccal mucosa. Marked weakness and paralysis occur in about 30% of episodes, which may lead to respiratory failure requiring mechanical ventilation. Crises typically last 1 to 7 days but recuperation from paralytic crises can be prolonged.
Renal involvement is manifested as a Fanconi-like syndrome with normal anion gap metabolic acidosis, hyperphosphaturia, hypophosphatemia, and vitamin D–resistant rickets. Nephromegaly and nephrocalcinosis may be present on ultrasound examination.
Hypertrophic cardiomyopathy and hyperinsulinism are seen in some infants.
Laboratory Findings
The presence of elevated levels of succinylacetone in serum and urine is diagnostic for tyrosinemia type I (see Fig. 79-1). In untreated patients, routinely available tests have a characteristic pattern. α-Fetoprotein level is increased, often markedly, and liver-synthesized coagulation factors are decreased in most patients; serum levels of transaminases are often increased, with marked increases being possible during acute hepatic episodes. Serum concentration of bilirubin is usually normal but can be increased with liver failure. Increased levels of α-fetoprotein are present in the cord blood of affected infants, indicating intrauterine liver damage. Plasma tyrosine level is usually elevated at diagnosis but this is a nonspecific finding and is dependent on dietary intake. Other amino acids, particularly methionine, may also be elevated in patients with liver damage. Hyperphosphaturia, hypophosphatemia, and generalized aminoaciduria may occur. The urinary level of 5-aminolevulinic acid is elevated (due to inhibition of 5-aminolevulinic hydratase by succinylacetone).
Diagnosis is usually established by demonstration of elevated levels of succinylacetone in urine or blood. Neonatal screening for hypertyrosinemia detects only a minority of patients with tyrosinemia type I. Succinylacetone, which is now assayed by most screening programs, has higher sensitivity and specificity than tyrosine and is the preferable metabolite for screening. Tyrosinemia type I should be differentiated from other causes of hepatitis and hepatic failure in infants, including galactosemia, hereditary fructose intolerance, neonatal iron storage disease, giant cell hepatitis, and citrullinemia type II (Chapter 79.11).
Treatment and Outcome
A diet low in phenylalanine and tyrosine can slow but does not halt the progression of the condition. The treatment of choice is nitisinone (NTBC), which inhibits tyrosine degradation at 4-HPPD (see Fig. 79-1). This treatment prevents acute hepatic and neurologic crises. Although nitisinone stops or greatly slows disease progression, some pretreatment liver damage is not reversible. Therefore, patients must be followed for development of cirrhosis or hepatocellular carcinoma. On imaging, the presence of even a single liver nodule usually indicates underlying cirrhosis. Most liver nodules in tyrosinemic patients are benign but current imaging techniques do not accurately distinguish all malignant nodules. Liver transplantation is an effective therapy for tyrosinemia type I and alleviates the risk of hepatocellular carcinoma. The impact of nitisinone treatment on the need for liver transplantation is still under study but the greatest effect is in patients treated early, such as children detected by neonatal screening, prior to the development of clinical symptoms. Rarely, nitisinone-treated patients develop corneal crystals, presumably of tyrosine, which are reversible by strict dietary compliance. This finding, combined with observations of developmental delay in some patients with chronically elevated tyrosine such as tyrosinemia type II, suggests that a diet low in phenylalanine and tyrosine should be continued in patients treated with nitisinone.
Genetics and Prevalence
Tyrosinemia type I is an autosomal recessive trait. The FAH gene maps to chromosome 15q; numerous mutations are reported. DNA analysis is useful for molecular prenatal diagnosis and for carrier testing in groups at risk for specific mutations such as French-Canadians from the Saguenay-Lac Saint-Jean region of Quebec. Tyrosinemia type I is panethnic; lack of French-Canadian or Scandinavian ancestry does not exclude the diagnosis. The prevalence of the condition is estimated to be 1/1,846 live births in the Saguenay-Lac Saint-Jean region and ≈1/100,000 worldwide. Prenatal diagnosis is typically performed by measurement of succinylacetone in amniotic fluid, or if the familial mutations are known, by DNA analysis of amniocytes or of chorionic villi.
Tyrosinemia Type II (Richner-Hanhart Syndrome, Oculocutaneous Tyrosinemia)
This rare autosomal recessive disorder is caused by deficiency of tyrosine aminotransferase, resulting in palmar and plantar hyperkeratosis, herpetiform corneal ulcers, and mental retardation (see Fig. 79-1). Ocular manifestations include excessive tearing, redness, pain, and photophobia and often occur before skin lesions. Corneal lesions are presumed to be due to tyrosine deposition. In contrast to herpetic ulcers, corneal lesions in tyrosinemia type II stain poorly with fluorescein and often are bilateral. Skin lesions, which may develop later in life, include painful, nonpruritic hyperkeratotic plaques on the soles, palms and fingertips. Mental retardation, which occurs in <50% of patients, is usually mild to moderate.
The principal laboratory finding in untreated patients is marked hypertyrosinemia (20-50 mg/dL; 1,100-2,750 µmole/L). Surprisingly, 4-hydroxyphenylpyruvic acid and its metabolites are also elevated in urine despite being downstream from the metabolic block (see Fig. 79-1). This is hypothesized to occur via the action of other transaminases in the presence of high tyrosine concentrations, producing 4HPP in cellular compartments like the mitochondrion in which it is not further degraded. In contrast to tyrosinemia type I, liver and kidney function are normal, as are serum concentrations of other amino acids and succinylacetone. Tyrosinemia type II is due to TAT gene mutations, causing deficiency of cytosolic tyrosine aminotransferase activity in liver.
Treatment with a diet low in tyrosine and phenylalanine improves the biochemical abnormalities and can normalize the skin and eye. The claim that mental retardation may be prevented by early diet therapy is reasonable and is supported by some case reports. The TAT gene maps to chromosome 16q and several disease-causing mutations have been identified. About half of reported cases are of Italian descent.
Tyrosinemia Type III (Primary Deficiency of 4-HPPD)
Only a few cases have been reported; most were detected by amino acid chromatography performed for various neurologic findings. Age at presentation has been from 1 to 17 mo. Developmental delay, seizures, intermittent ataxia, and self-destructive behavior are reported. A causal link to 4HPP deficiency is not formally established. Liver or renal abnormalities are absent. Asymptomatic infants with 4-HPPD deficiency have been identified by neonatal screening for hypertyrosinemia.
The diagnosis is suspected in children with sustained moderate increases in plasma levels of tyrosine (typically 350-700 µmole/L on a normal diet) and the presence in urine of 4-hydroxyphenylpyruvic acid and its metabolites 4-hydroxyphenyllactic and 4-hydroxyphenylacetic acids. Diagnosis may be refined by demonstrating the presence of mutations in the gene for 4-HPPD on chromosome 12q, or rarely, by demonstrating a low activity of 4-HPPD enzyme in liver biopsy.
Given the possible association with neurologic abnormalities, dietary reduction of plasma tyrosine levels is prudent. It is also logical to attempt a trial of vitamin C, the cofactor for 4-HPPD. The condition is inherited as an autosomal recessive trait.
Transient Tyrosinemia of the Newborn
In a small number of newborn infants, plasma tyrosine may be as high as 60 mg/dL (3,300 µmole/L) during the 1st 2 weeks of life. Most affected infants are premature and are receiving high-protein diets. Transient tyrosinemia is felt to result from delayed maturation of 4-HPPD (see Fig. 79-1). Lethargy, poor feeding, and decreased motor activity are noted in some patients. Most are asymptomatic and are identified by a high blood phenylalanine or tyrosine level on screening. Laboratory findings include marked elevation of plasma tyrosine with a moderate increase in plasma phenylalanine. The finding of hypertyrosinemia differentiates this condition from PKU. 4-Hydroxyphenylpyruvic acid and its metabolites are present in the urine. Hypertyrosinemia usually resolves spontaneously in the 1st mo of life. It can be corrected promptly by reducing dietary protein to below 2 g/kg/24 hr and by administering vitamin C (200-400 mg/24 hr). Mild intellectual deficits have been reported in some infants that had this condition, but the causal relationship to hypertyrosinemia is not conclusively established.
Hawkinsinuria
This rare autosomal dominant condition is caused by a mutant 4-HPPD enzyme that catalyzes a partial reaction, releasing an intermediate compound used for diagnosis (see Fig. 79-1). This intermediate is either reduced to form 4-hydroxycyclohexylacetic acid (4-HCAA) or reacts with glutathione to form the unusual organic acid hawkinsin (2-L-cysteine-S-yl-1-4-dihydroxycyclohex-5-en-1-yl-acetic acid); secondary glutathione deficiency may occur.
Affected children and adults excrete the organic acids 4-HCAA, 4-hydroxyphenylpyruvic acid and its metabolites (4-hydroxyphenyllactic and 4-hydroxyphenylacetic acids), 5-oxoproline (owing to secondary glutathione deficiency), and hawkinsin in their urine. Plasma tyrosine level is usually normal.
Treatment consists of a low-protein diet during infancy. Breast feeding is encouraged. A trial with large doses of vitamin C (up to 1,000 mg/24 hr) is also recommended. The same mutation, a substitution of threonine for the normal alanine codon at position 33 of the 4-HPPD gene, has been identified in unrelated patients with hawkinsinuria.
Alcaptonuria
This rare (with an incidence of ≈1/250,000) autosomal recessive disorder is due to a deficiency of homogentisic acid oxidase, which causes large amounts of homogentisic acid to accumulate in the body and then to be excreted in the urine (see Fig. 79-1).
The diagnosis is confirmed by finding massive excretion of homogentisic acid on urine organic acid testing. Tyrosine levels are normal. The enzyme is expressed only in the liver and kidneys. The gene for alcaptonuria, HGD, maps to chromosome 3q. Several disease-causing mutations have been identified. Alcaptonuria is commonest in the Dominican Republic and Slovakia.
Treatment of the arthritis is symptomatic. Nitisinone efficiently reduces homogentisic acid production in alkaptonuria. If presymptomatic individuals are detected, treatment with nitisinone, combined with a phenylalanine- and tyrosine-restricted diet, seems reasonable, although no experience is available regarding long-term efficacy.
Albinism
Albinism is due to deficiency of melanin, the main pigment of the skin and eye (Table 79-1). Melanin is synthesized by melanocytes from tyrosine in a membrane-bound intracellular organelle, the melanosome. Melanocytes originate from the embryonic neural crest and migrate to the skin, eyes (choroid and iris), hair follicles, and inner ear. The melanin in the eye is confined to the retinal pigment epithelium, whereas in skin and hair follicles, it is secreted into the epidermis and hair shaft. Albinism can be caused by deficiencies of melanin synthesis, by some hereditary defects of melanosomes, or by disorders of melanocyte migration. Although albinism is a classical example of a biochemical genetic disease, neither the biosynthetic pathway of melanin nor many facets of melanocyte cell biology are completely elucidated (see Fig. 79-2). The end products are 2 pigments: pheomelanin, which is a yellow-red pigment; and eumelanin, a brown-black pigment.
Table 79-1 CLASSIFICATION OF ALBINISM
TYPE | GENE | CHROMOSOME |
---|---|---|
OCULOCUTANEOUS ALBINISM (OCA) | ||
OCA1 (tyrosinase deficient) | TYR | 11q |
OCA1A (severe deficiency) | TYR | 11q |
OCA1B (mild deficiency)* | TYR | 11q |
OCA2 (tyrosinase positive)† | P (pink-eyed dilution) | 15q |
OCA3 (Rufous, red OCA) | TYRP1‡ | 9p |
OCA4 | MATP | 5p13.3 |
Hermansky-Pudlak syndrome | HPS1 | 10q |
Chédiak-Higashi syndrome | LYST | 1q |
OCULAR ALBINISM | ||
OA1 (Nettleship-Falls type) | OA | xp |
LOCALIZED ALBINISM | ||
Piebaldism | KIT | 4q |
Waardenburg syndrome I & III | PAX3 | 2q |
Waardenburg syndrome II | MITF | 3p |
* This includes Amish, minimal pigment, yellow albinism, and platinum and temperature-sensitive variants.
‡ Tyrosinase related protein 1.
Clinically, primary albinism can be generalized or localized. Primary generalized albinism can be either ocular or oculocutaneous. Some syndromes feature albinism in association with platelet, immunological, or neurological dysfunction.
In generalized oculocutaneous albinism, hypopigmentation can be either complete or partial. Individuals with complete albinism do not develop detectable skin pigmentation, either generalized (tanning) or localized (pigmented nevi).
The diagnosis of albinism is usually evident, but for some white children whose families are particularly light-skinned, normal variation may be a diagnostic consideration. Such normal fair-skinned children progressively develop pigmentation, the eye manifestations of albinism are absent, and other family members may have had a similar course. The clinical diagnosis of oculocutaneous albinism, as opposed to other types of cutaneous hypopigmentation, requires the presence of characteristic eye findings.
The ocular manifestations of albinism include hypopigmentation, including foveal hypoplasia with reduced visual acuity, refractive errors, nystagmus, alternating strabismus, and a red reflex (diffuse reddish hue of the iris produced during ophthalmoscopic or slit lamp examination of the eye). There is also an abnormality in routing of the optic fibers at the chiasm. Unlike normally pigmented individuals, in patients with albinism the majority of the nerve fibers from the temporal side of the retina cross to the contralateral hemisphere of the brain. This results in lack of biocular (stereoscopic) vision and of depth perception, and in repeated switching of vision from eye to eye, causing alternating strabismus. This abnormality also causes a characteristic pattern of visual evoked potentials. These findings are highly specific for albinism and can be used to formally establish the clinical diagnosis. Regular ophthalmological follow-up is recommended for patients with albinism. For instance, correction of refractive errors can maximize visual function. Normally the alternating strabismus does not result in amblyopia and does not require surgery.
Patients with albinism should be counseled to avoid ultraviolet radiation by wearing protective long-sleeved clothing and by using sunscreens with a sun protection factor (SPF) rating above 30. All forms of oculocutaneous albinism are autosomal recessive traits.
Melanin is also present in the cochlea. Albino individuals may be more susceptible to ototoxic agents such as gentamicin.
Many clinical forms of albinism have been identified. Some of the seemingly distinct clinical forms are caused by different mutations of the same gene. Several genes located on different chromosomes are shown to be involved in melanogenesis (see Table 79-1). Attempts to differentiate types of albinism based on the mode of inheritance, tyrosinase activity, or the extent of hypopigmentation have failed to yield a comprehensive classification. The following classification is based on the distribution of albinism in the body and the type of mutated gene.
Mutation detection is clinically available for most albinism genes (see Table 79-1). Molecular diagnosis is of little use therapeutically in isolated albinism but can be helpful for precise genetic counseling of families.
Oculocutaneous (Generalized) Albinism (OCA)
Lack of pigment is generalized, affecting skin, hair, and eyes. Three genetically distinct forms exist: OCA1, OCA2 and OCA3. The lack of pigment is complete in patients with OCA1 A; the other types may not be clinically distinguishable from one another. All are inherited as autosomal recessive traits.
OCA1 (Tyrosinase-Deficient Albinism)
The defect in these patients resides in the tyrosinase gene, TYR, on chromosome 11q. Many mutant alleles have been identified. Most affected individuals are genetic compounds, heterozygous for 2 different mutant alleles. A clinical clue to the diagnosis of OCA1 is complete lack of pigment at birth. The condition can be subdivided to OCA1 A and OCA1 B, based on enzyme activity and later clinical manifestations.
OCA1 A (Tyrosinase-Negative OCA)
In these individuals, both TYR alleles have mutations that completely inactivate tyrosinase. Clinically, lack of pigment in the skin (milky white), hair (white hair), and eyes (red gray irides) is evident at birth and remains unchanged throughout life. They do not tan and do not develop pigmented nevi or freckles.
OCA1 B
These patients have TYR gene mutations that preserve some residual activity. Clinically they completely lack pigment at birth, but with age become light blond with light blue or hazel eyes. They develop pigmented nevi and freckles and they may tan. OCA1 B patients, depending on the degree of pigmentation, were once subdivided into different groups and thought to be genetically distinct.
OCA2 (Tyrosinase-Positive OCA)
This is the most common form of generalized OCA, particularly in African blacks. Clinically, patients demonstrate some pigmentation of the skin and eyes at birth and continue to accumulate pigment throughout their lives. The hair is yellow at birth and may darken with age. They have pigmented nevi and freckles but do not tan. They may be clinically indistinguishable from OCA1 B. These individuals have normal tyrosinase activity in hair bulbs. The defect is in the OCA2 gene on chromosome 15q, orthologous to the p (pink-eyed dilution) gene in the mouse. This gene produces the P protein, a melanosome membrane protein. Patients with Prader-Willi and Angelman syndromes with microdeletion in chromosome 15q12 lack 1 copy of the OCA2 gene and have mild pigmentary dilution (Chapter 76).
OCA3 (Rufous Albinism)
This form has been identified only in Africans, African-Americans, and natives of New Guinea. Patients have reddish hair and reddish brown skin as adults. The skin color is peculiar to this form. In the young, the coloration may resemble that of OCA2. Patients with OCA3 can make pheomelanin but not eumelanin. The mutation is in the tyrosinase related protein 1 (TYRP1) gene, the function of which is not understood.
Ocular Albinism (OA)
Albinism is limited to the eye. All the eye findings of albinism (see earlier) are present. Most cases are X-linked (OA1). In pedigrees with apparently autosomal recessive OA, OCA2 with predominant ocular involvement should be considered.
Ocular Albinism 1 (OA1 Nettleship-Falls Type)
Only the hemizygous male has the complete manifestation. Segments of abnormal retinal pigmentation may be present in heterozygous females. An X-linked ocular albinism with late-onset sensorineural deafness has also been reported. The diagnosis of OA1 is evident in males with the features of albinism in the eye, normal skin pigmentation, and a positive family history suggestive of an X-linked recessive transmission. In patients who are the 1st of their families to be affected, electron microscopic demonstration of characteristic megamelanosomes in skin biopsies or hair root specimens is useful, as is mutation analysis of the OA1 gene on chromosome Xp.
Syndromic Forms of Generalized Albinism
Hermansky-Pudlak Syndrome
This group of autosomal recessive disorders is caused by mutations of 1 of 8 genes, HPS1 to HPS8. Hermansky-Pudlak syndrome is suspected in patients with albinism and a bleeding diathesis. Disease subtype can be established with molecular studies.
The HPS genes are necessary for normal structure and function of lysosome-derived organelles, including melanosomes and platelet dense bodies. Patients have a tyrosinase-positive OCA of variable severity associated with platelet dysfunction (owing to the absence of platelet dense bodies). A ceroid-like material accumulates in tissues. Hermansky-Pudlak syndrome is most prevalent in 2 regions of Puerto Rico (types 1 and 3, due to different founder effects). The cutaneous and ocular symptoms of albinism are present. Patients can develop epistaxis, postsurgical bleeding, or abundant menses. Bleeding time is prolonged but platelet count is normal. Major complications are progressive pulmonary fibrosis in young adults and Crohn’s-like inflammatory bowel disease in adolescents and young adults. Kidney failure and cardiomyopathy are reported. Neutropenia is described in HPS2. Treatment is symptomatic.
Chédiak-Higashi Syndrome
Patients with this rare autosomal recessive condition (Chapter 124) have albinism of variable severity and susceptibility to infection. Bacterial infections of skin and upper respiratory tract are common. Giant peroxidase-positive lysosomal granules can be seen in granulocytes in a blood smear. Patients have a reduced number of melanosomes, which are abnormally large (macromelanosomes). The bleeding tendency is typically mild. The major, life-threatening complication is macrophage activation with hemophagocytic lymphohistiocytosis, manifested by fever, lymphadenopathy, hepatosplenomegaly, cytopenias, and elevated plasma ferritin level. Patients surviving childhood may develop cerebellar atrophy, peripheral neuropathy, and cognitive delay. Mutations in the LYST gene on chromosome 1q are the only known cause of this syndrome.
Localized Albinism
This term refers to localized patches of hypopigmentation of skin and hair, which may be evident at birth or develop with time. These conditions are caused by abnormal migration of melanocytes during development.
Piebaldism
In this autosomal dominant inherited condition, the individual is usually born with a white forelock. The underlying skin is depigmented and devoid of melanocytes. In addition, there are usually white macules on the face, trunk, and extremities. Mutations in the KIT gene have been shown in affected patients.
Waardenburg Syndrome
In this syndrome, a white forelock is associated with lateral displacement of inner canthi, broad nasal bridge, heterochromia of irides, and sensorineural deafness. This condition is inherited as an autosomal dominant trait. Four major types of this syndrome have been identified. Patients with type I have lateral displacement of inner canthi. The condition is caused by mutations in the PAX3 gene. Type II patients have normal inner canthal distances, and mutations in the MITF gene have been shown in some patients. Patients with type III have all the findings seen in individuals with type I, plus hypoplasia and contractures of the upper limbs. The gene abnormality is in PAX3. Type IV, associated with Hirschsprung disease, is heterogeneous. Mutations in different genes (EDN3, EDNRB, or SOX10) have been identified in different patients.
Other causes of localized hypopigmentation, such as somatic mosaicism for chromosomal abnormalities are dealt with elsewhere (e.g., hypomelanosis of Ito, Chapters 76 and 645; and vitiligo, Chapter 645).
Brilliant MH. Oculocutaneous albinism type 4, 2007. GeneReviews at GeneTests: Medical Genetics Information Resources. University of Washington, Seattle, 1997-2010. www.genetests.org. Accessed June 2010
Charfeddine C, Monastiri K, Mokni M, et al. Clinical and mutational investigations of tyrosinemia type II in Northern Tunisia: identification and structural characterization of two novel TAT mutations. Mol Genet Metab. 2006;88:184-191.
El-Karaksy H, Rashed M, El-Sayed R, et al. Clinical practice. NTBC therapy for tyrosinemia type 1: how much is enough? Eur J Pediatr. 2010;169:689-693.
Gahl WA. Hermansky-Pudlak syndrome, 2010. GeneReviews at GeneTests: Medical Genetics Information Resources. University of Washington, Seattle, 1997-2010. www.genetests.org. Accessed June 2010
Introne WJ, Westbroek W, Golas GA, et al. Chédiak-Higashi syndrome, 2009. GeneReviews at GeneTests: Medical Genetics Information Resources. University of Washington, Seattle, 1997-2010. www.genetests.org. Accessed May 2010
King RA, Oetting W. Oculocutaneous albinism type 2, 2007. GeneReviews at GeneTests: Medical Genetics Information Resources. University of Washington, Seattle, 1997-2010. www.genetests.org. Accessed June 2010
Masurel-Paulet A, Poggi-Bach J, Rolland MO, et al. NTBC treatment in tyrosinaemia type I: long-term outcome in French patients. J Inherit Metab Dis. 2008;31:81-87.
Pingault V, Ente D, Dastot-LeMoal F, et al. Review and update of mutations causing Waardenburg syndrome. Hum Mutat. 2010;31:391-406.
Rezvani I. 50 years ago in Journal of Pediatrics: Waardenburg’s syndrome. J Pediatr. 2010;157:732.
Scott CR. The genetic tyrosinemias. Am J Med Genet C Semin Med Genet. 2006;142C:121-126.
79.3 Methionine
The normal pathway for catabolism of methionine, an essential amino acid, produces S-adenosylmethionine, which serves as a methyl group donor for methylation of a variety of compounds in the body, and cysteine, which is formed through a series of reactions collectively called trans-sulfuration (Fig. 79-3).
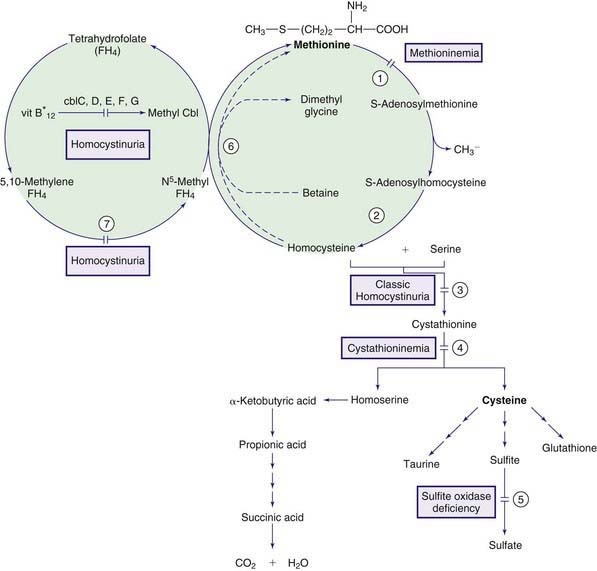
Figure 79-3 Pathways in the metabolism of sulfur-containing amino acids. Enzymes: (1) methionine adenosyltransferase (MAT I/III), (2) adenosylhomocysteine hydrolase, (3) cystathionine synthase, (4) cystathionase, (5) sulfite oxidase, (6) betaine homocysteine methyltransferase, (7) methylene tetrahydrofolate reductase.
Homocystinuria (Homocystinemia)
Normally, most homocysteine, an intermediate compound of methionine degradation, is remethylated to methionine. This methionine-sparing reaction is catalyzed by the enzyme methionine synthase, which requires a metabolite of folic acid (5-methyltetrahydrofolate) as a methyl donor and a metabolite of vitamin B12 (methylcobalamin) as a cofactor (see Fig. 79-3). Only 20-30% of total homocysteine (and its dimer homocystine) is in free form in the plasma of normal individuals. The rest is bound to proteins as mixed disulfides. Three major forms of homocystinemia and homocystinuria have been identified.
Homocystinuria Due to Cystathionine β-Synthase (CBS) Deficiency (Classic Homocystinuria)
This is the most common inborn error of methionine metabolism. About 40% of affected patients respond to high doses of vitamin B6 and usually have milder clinical manifestations than those who are unresponsive to vitamin B6 therapy. These patients possess some residual enzyme activity.
Infants with this disorder are normal at birth. Clinical manifestations during infancy are nonspecific and may include failure to thrive and developmental delay. The diagnosis is usually made after 3 yr of age, when subluxation of the ocular lens (ectopia lentis) occurs. This causes severe myopia and iridodonesis (quivering of the iris). Astigmatism, glaucoma, staphyloma, cataracts, retinal detachment, and optic atrophy may develop later in life. Progressive mental retardation is common. Normal intelligence has been reported. In an international survey of >600 patients, IQ scores ranged from 10 to 135. Higher IQ scores are seen in vitamin B6 responsive patients. Psychiatric and behavioral disorders have been observed in >50% of affected patients. Convulsions occur in about 20% of patients. Affected individuals with homocystinuria manifest skeletal abnormalities resembling those of Marfan syndrome (Chapter 693); they are usually tall and thin, with elongated limbs and arachnodactyly. Scoliosis, pectus excavatum or carinatum, genu valgum, pes cavus, high arched palate, and crowding of the teeth are commonly seen. These children usually have fair complexions, blue eyes, and a peculiar malar flush. Generalized osteoporosis, especially of the spine, is the main roentgenographic finding. Thromboembolic episodes involving both large and small vessels, especially those of the brain, are common and may occur at any age. Optic atrophy, paralysis, cor pulmonale, and severe hypertension (due to renal infarcts) are among the serious consequences of thromboembolism, which is caused by changes in the vascular walls and increased platelet adhesiveness secondary to elevated homocystine levels. The risk of thromboembolism increases after surgical procedures. Spontaneous pneumothorax and acute pancreatitis are rare complications.
Treatment with high doses of vitamin B6 (200-1,000 mg/24 hr) causes dramatic improvement in most patients who are responsive to this therapy. The degree of response to vitamin B6 treatment may be different in different families. Some patients may not respond because of folate depletion; a patient should not be considered unresponsive to vitamin B6 until folic acid (1-5 mg/24 hr) has been added to the treatment regimen. Restriction of methionine intake in conjunction with cysteine supplementation is recommended for patients who are unresponsive to vitamin B6. The need for dietary restriction and its extent remains controversial in patients with vitamin B6 responsive form. In some patients with this form, addition of betaine may obviate the need for any dietary restriction. Betaine (trimethylglycine, 6-9 g/24 hr for adults or 200-250 mg/kg/day for children) lowers homocysteine levels in body fluids by remethylating homocysteine to methionine (see Fig. 79-3); this may result in further elevation of plasma methionine levels. This treatment has produced clinical improvement (preventing vascular events) in patients who are unresponsive to vitamin B6 therapy. Cerebral edema has occurred in a patient with vitamin B6 nonresponsive homocystinuria and dietary noncompliance during betaine therapy. Administration of large doses of vitamin C (1 g/day) has improved the endothelial function; long-term clinical efficacy is not known.
The screening of newborn infants for classic homocystinuria has been performed worldwide and a prevalence of 1/200,000 to 1/350,000 has been estimated. The condition seems more common in New South Wales, Australia (1/60,000), and Ireland. Early treatment of patients identified by the screening process has produced favorable results. The mean IQ of 16 patients with vitamin B6 unresponsive form treated in early infancy was 94 ± 4. Dislocation of the lens seemed to be prevented in some patients.
Homocystinuria is inherited as an autosomal recessive trait. The gene for cystathionine β-synthase is located on chromosome 21q22.3. Prenatal diagnosis is feasible by performing an enzyme assay of cultured amniotic cells or chorionic villi or by DNA analysis. Many disease-causing mutations have been identified in different families. The majority of affected patients are compound heterozygotes for 2 different alleles. Heterozygous carriers are usually asymptomatic; thromboembolic events and coronary heart disease are more common in these individuals than in the normal population.
Homocystinuria Due to Defects in Methylcobalamin Formation
Methylcobalamin is the cofactor for the enzyme methionine synthase, which catalyzes remethylation of homocysteine to methionine. There are at least 5 distinct defects in the intracellular metabolism of cobalamin that may interfere with the formation of methylcobalamin. To better understand the metabolism of cobalamin, see methylmalonic acidemia (Fig. 79-4; Chapter 79.6 and Fig. 79-3). The 5 defects are designated as cblC, cblD (including cblD variant 1), cblE (methionine synthase reductase), cblG (methionine synthase), and cblF. Patients with cblC, cblD (not those with cblD variant 2), and cblF defects have methylmalonic acidemia in addition to homocystinuria because formation of both adenosylcobalamin and methylcobalamin is impaired (Chapter 79.6).
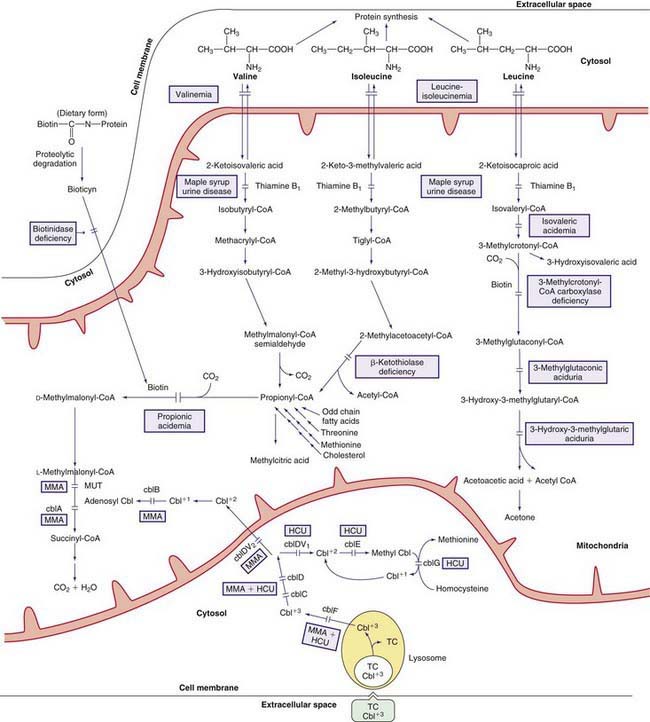
Figure 79-4 Pathways in the metabolism of the branched-chain amino acids, biotin, and vitamin B12 (cobalamin). MMA, methylmalonic acidemia; HCU, homocystinuria; Cbl, cobalamin; OHCbl, hydroxycobalamin; cbl, defect in metabolism of cobalamin; cblDV1, cblD variant 1; cblDV2, cblD variant 2; TC, transcobalamin.
Patients with cblE and cblG defects are unable to form methylcobalamin and develop homocystinuria without methylmalonic acidemia (see Fig. 79-4); fewer than 40 patients are known with each of these diseases.
Diagnosis is established by complementation studies performed in cultured fibroblasts. Prenatal diagnosis has been accomplished by studies in amniotic cell cultures. All of these conditions are inherited as autosomal recessive traits. The gene for cblE (MTRR) is on chromosome 5p15.3-p15.2 and that for cblG (MTR) is on chromosome 1q43; several disease-causing mutations, including a common missense mutation (P1173L) in the MTR gene, have been described.
Treatment with vitamin B12 in the form of hydroxycobalamin (1-2 mg/24 hr) is used to correct the clinical and biochemical findings. Results vary among both diseases and sibships.
Homocystinuria Due to Deficiency of Methylenetetrahydrofolate Reductase (MTHFR)
This enzyme reduces 5,10-methylenetetrahydrofolate to form 5-methyltetrahydrofolate, which provides the methyl group needed for remethylation of homocysteine to methionine (see Fig. 79-3).
Laboratory findings include moderate homocystinemia and homocystinuria. The methionine concentration is low or low normal. This finding differentiates this condition from classic homocystinuria caused by cystathionine synthase deficiency. Absence of megaloblastic anemia distinguishes this condition from homocystinuria caused by methylcobalamin formation (see earlier). Thromboembolism of vessels has also been observed in these patients. Diagnosis may be confirmed by the enzyme assay in cultured fibroblasts or leukocytes or by finding causal mutation in the MTHR gene.
A number of polymorphisms have been described in the MTHR gene. Two of these (677C → T and 1298A → C) may affect levels of plasma total homocysteine and have been studied as possible risk factors for a wide variety of medical conditions, ranging from birth defects to vascular disease and even cancer, Alzheimer disease, and death from leukemia. To date, the best data support a role for 677C → T polymorphism as a risk factor for neural tube defects. Although a clinical test for this polymorphism is widely available, its predictive value in any given individual has yet to be determined.
Treatment of severe MTHFR deficiency with a combination of folic acid, vitamin B6, vitamin B12, methionine supplementation, and betaine has been tried. Of these, early treatment with betaine seems to have the most beneficial effect.
The condition is inherited as an autosomal recessive trait; the gene for the enzyme has been located on chromosome 1p36.3 and many disease-causing mutations have been reported. Prenatal diagnosis can be offered by measuring MTHFR enzyme activity in cultured chorionic villi cells or amniocytes, by linkage analysis in informative families, or by DNA analysis of the mutation.
Hypermethioninemia
Secondary hypermethioninemia occurs in liver disease, tyrosinemia type I, and classic homocystinuria. Hypermethioninemia has also been found in premature and some full-term infants receiving high-protein diets, in whom it may represent delayed maturation of the enzyme methionine adenosyltransferase. Lowering the protein intake usually resolves the abnormality. Primary hypermethioninemia caused by the deficiency of hepatic methionine adenosyltransferase (MAT I/III; MAT II, which is present in other tissues, is not affected; see Fig. 79-3) has been reported in approximately 60 patients. The majority of these patients have been diagnosed in the neonatal period through screening for homocystinuria. Affected individuals with residual enzyme activity remain asymptomatic throughout life despite persistent hypermethioninemia. Some complain of unusual odor to their breath (boiled cabbage). A few patients with complete enzyme deficiency have had neurologic abnormalities related to demyelination (mental retardation, dystonia, dyspraxia). Normal pregnancies producing normal offspring have been reported in mothers with methionine adenoslytransferase deficiency. The condition is inherited as an autosomal recessive trait. The gene for hepatic methionine adenosyltransferase is on chromosome 10q22 and several disease-causing mutations have been identified. A novel defect, glycine N-methyltransferase deficiency, also causes isolated hypermethioninemia.
Cystathioninemia (Cystathioninuria)
Secondary cystathioninuria occurs in patients with vitamin B6 or B12 deficiency, liver disease (particularly damage caused by galactosemia), thyrotoxicosis, hepatoblastoma, neuroblastoma, ganglioblastoma, or defects in remethylation of homocysteine.
Cystathionase deficiency results in massive cystathioninuria and mild to moderate cystathioninemia; cystathionine is not normally detectable in blood. Deficiency of this enzyme is inherited as an autosomal recessive trait and its prevalence is estimated to be about 1/14,000 live births. Affected subjects with a wide variety of clinical manifestations have been reported. Lack of a consistent clinical picture and the presence of cystathioninuria in a number of normal persons suggest that cystathionase deficiency may be of no clinical significance. A majority of reported cases are responsive to oral administration of large doses of vitamin B6 (≥100 mg/24 hr). When cystathioninuria is discovered in a patient, vitamin B6 treatment seems indicated, but its beneficial effect has not been established. The gene encoding for cystathionase is located on chromosome 16.
Adams D, Venditti CP. Disorders of intracellular cobalamin metabolism. GeneReviews at GeneTests: Medical Genetics Information Resources. University of Washington, Seattle, 1997-2010. www.genetests.org. Accessed May 2010
Allen NC, Bagade S, McQueen MB, et al. Systematic meta-analyses and field synopsis of genetic association studies in schizophrenia: the SzGene database. Nat Genet. 2008;30:827-834.
Forges T, Chery C, Audonnet S, et al. Life-threatening methylenetetrahydrofolate reductase (MTHFR) deficiency with extremely early onset: characterization of two novel mutations in compound heterozygous patients. Mol Genet Metab. 2010;100:143-148.
Holm PK, Hustad S, Ueland PM, et al. Modulation of the homocysteine-betaine relationship by methylenetetrahydrofolate reductase 677C-T genotypes and B-vitamin status in a large-scale epidemiologic study. J Clin Endocr Metab. 2007;92:1535-1541.
Lawson-Yuen A, Levy HL. The use of betaine in the treatment of elevated homocysteine. J Mol Genet Metab. 2006;88:201-207.
Lin HJ, Neidich JA, Salazar D, et al. Asymptomatic maternal combined homocystinuria and methylmalonic aciduria (cbIC) detected through low carnitine levels on newborn screening. J Pediatr. 2009;155:924-927.
Picker JD, Levy HL. Homocystinuria caused by cystathionine beta-synthase deficiency, 2006. GeneReviews at GeneTests: Medical Information Resource. University of Washington, Seattle, 1997-2010. www.genetests.org. Accessed May 2010
Schiff M, Benoist JF, Tilea B, et al. Isolated remethylation disorders: do our treatments benefit patients? J Inherit Metab Dis. 2011;34:137-145.
Skovby F, Gaustadnes M, Mudd SH. A revisit to the natural history of homocystinuria due to cystathionine beta-synthase deficiency. Mol Genet Metab. 2010;99:1-3.
Strauss KA, Morton DH, Puffenberger EG, et al. Prevention of brain disease from severe 5,10-methylenetetrahydrofolate reductase deficiency. Mol Genet Metab. 2007;91:165-175.
Testai FD, Gorelick PB. Inherited metabolic disorders and stroke part 2: homocystinuria, organic acidurias, and urea cycle disorders. Arch Neurol. 2010;67:148-153.
79.4 Cysteine/Cystine
Cysteine is a sulfur-containing nonessential amino acid that is synthesized from methionine (see Fig. 79-3). In the presence of oxygen, 2 molecules of cysteine are oxidized to form cystine. The most common disorders of cysteine/cystine metabolism, cystinuria (Chapter 541) and cystinosis (Chapter 523.3).
Sulfite Oxidase Deficiency (Molybdenum Cofactor Deficiency)
At the last step in cysteine metabolism, sulfite is oxidized to sulfate by sulfite oxidase, and the sulfate is excreted in the urine (see Fig. 79-3). This enzyme requires a molybdenum-pterin complex named molybdenum cofactor. This cofactor is also necessary for the function of 2 other enzymes in humans: xanthine dehydrogenase (which oxidizes xanthine and hypoxanthine to uric acid) and aldehyde oxidase. Three enzymes, encoded by 3 different genes, are involved in the synthesis of the cofactor. The genes are mapped to chromosomes 14q24, 6p21.3, and 5q11. Deficiency of any of the 3 enzymes causes cofactor deficiency with identical phenotype. Most patients who were originally diagnosed as having sulfite oxidase deficiency have been proven to have molybdenum cofactor deficiency. Both conditions are inherited as autosomal recessive traits. The gene for sulfite oxidase is on chromosome 12.
These children excrete large amounts of sulfite, thiosulfate, S-sulfocysteine, xanthine, and hypoxanthine in their urine. Urinary and serum levels of uric acid and urinary concentration of sulfate are diminished. Fresh urine should be used for screening purposes and for quantitative measurements of sulfite, because oxidation at room temperature may produce false-negative results.
Diagnosis is confirmed by measurement of sulfite oxidase and molybdenum cofactor in fibroblasts and liver biopsies, respectively. Prenatal diagnosis is possible by performing an assay of sulfite oxidase activity in cultured amniotic cells or in samples of chorionic villi.
No effective treatment is available, and most children die in the 1st 2 yr of life. The prevalence of these deficiencies in the general population is not known.
Kugler S, Hahnewald R, Garrido M, et al. Long-term rescue of a lethal inherited disease by adeno-associated virus-mediated gene transfer in a mouse model of molybdenum-cofactor deficiency. Am J Hum Genet. 2007;80:291-297.
Schwarz G, Mendel RR, Ribbe MW. Molybdenum cofactors, enzymes and pathways. Nature. 2009;460:839-847.
Tan WH, Eickler FS, Hoda S, et al. Isolated sulfite oxidase deficiency: a case report with a novel mutation and review of the literature. Pediatrics. 2005;116:757-766.
Veldman A, Santamaria-Araujo JA, Sollazzo S, et al. Successful treatment of molybdenum cofactor deficiency type A with cPMP. Pediatrics. 2010;125:e1249-e1254.
79.5 Tryptophan
Tryptophan is an essential amino acid and a precursor for nicotinic acid (niacin) and serotonin (Fig. 79-5). The genetic disorders of metabolism of serotonin, 1 of the major neurotransmitters, are discussed in Chapter 79.11.
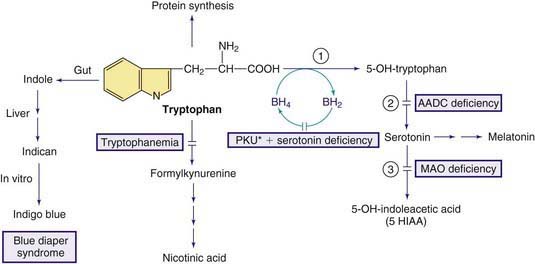
Figure 79-5 Pathways in the metabolism of tryptophan. PKU* indicates hyperphenylalanemia due to tetrahydrobiopterin deficiency (see Fig. 79-1). Enzymes: (1) tryptophan hydroxylase, (2) aromatic L-amino acid decarboxylase (AADC), (3) monoamine oxidase (MAO).
Hartnup Disorder
In this autosomal recessive disorder, named after the 1st affected family to be reported, there is a defect in the transport of monoamino-monocarboxylic amino acids (neutral amino acids), including tryptophan, by the intestinal mucosa and renal tubules. Decreased intestinal absorption of tryptophan in conjunction with its increased renal loss is believed to cause reduced availability of tryptophan for niacin synthesis. Most children with Hartnup defect remain asymptomatic. The major clinical manifestation in the rare symptomatic patient is cutaneous photosensitivity. The skin becomes rough and red after moderate exposure to the sun, and with greater exposure, a pellagra-like rash may develop. The rash may be pruritic, and a chronic eczema may appear. The skin changes have been reported in affected infants as young as 10 days of age. Some patients may have intermittent ataxia manifested as an unsteady, wide-based gait. The ataxia may last a few days and usually recovers spontaneously. Mental development is usually normal. Two individuals in the original kindred were mentally retarded. Episodic psychologic changes, such as irritability, emotional instability, depression, and suicidal tendencies, have been observed; these changes are usually associated with bouts of ataxia. Short stature and atrophic glossitis are seen in some patients.
Most children diagnosed with Hartnup disorder by neonatal screening have remained asymptomatic. This indicates that other factors are also involved in pathogenesis of the clinical condition.
The main laboratory finding is aminoaciduria, which is restricted to neutral amino acids (alanine, serine, threonine, valine, leucine, isoleucine, phenylalanine, tyrosine, tryptophan, histidine). Urinary excretion of proline, hydroxyproline, and arginine remains normal. This finding differentiates Hartnup disorder from other causes of generalized aminoaciduria, such as Fanconi syndrome. Plasma concentrations of neutral amino acids are usually normal. This seemingly unexpected finding is because these amino acids are absorbed as dipeptides and the transport system for small peptides is intact in Hartnup disorder. The indole derivatives (especially indican) may be found in large amounts in some patients, owing to bacterial breakdown of unabsorbed tryptophan in the intestines.
Diagnosis is established by the striking intermittent nature of symptoms and the just described urinary findings.
Treatment with nicotinic acid or nicotinamide (50-300 mg/24 hr) and a high-protein diet results in a favorable response in symptomatic patients. Because of the intermittent nature of the clinical manifestations, the efficacy of these treatments is difficult to evaluate. The prevalence of the disorder is estimated to be 1/20,000 to 1/30,000. Normal outcome both for mother and fetus is reported in affected pregnant women. The gene (SLC6A19) for this condition is on chromosome 5p15.33.
79.6 Valine, Leucine, Isoleucine, and Related Organic Acidemias
The early steps in the degradation of these 3 essential amino acids, the branched-chain amino acids, are similar (see Fig. 79-4). The intermediate metabolites are all organic acids, and deficiency of any of the degradative enzymes, except for the transaminases, causes acidosis; in such instances, the organic acids before the enzymatic block accumulate in body fluids and are excreted in the urine. These disorders commonly cause metabolic acidosis, which usually occurs in the 1st few days of life. Although most of the clinical findings are nonspecific, some manifestations may provide important clues to the nature of the enzyme deficiency. An approach to infants suspected of having an organic acidemia is presented in Figure 79-6. Definitive diagnosis is usually established by identifying and measuring specific organic acids in body fluids (blood, urine), by the enzyme assay, and by identification of the mutant gene.
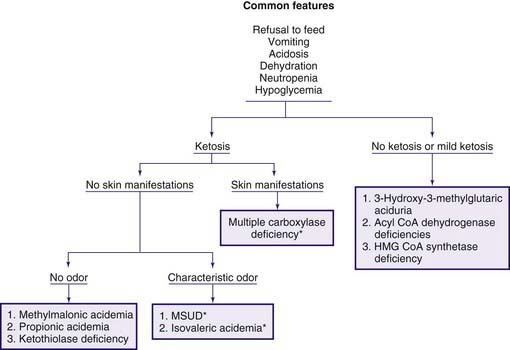
Figure 79-6 Clinical approach to infants with organic acidemia. Asterisks indicate disorders in which patients have a characteristic odor (see text and Table 79-2). MSUD, maple syrup urine disease.
Organic acidemias are not limited to defects in the catabolic pathways of branched-chain amino acids. Disorders causing accumulation of other organic acids include those derived from lysine (Chapter 79.14), those associated with lactic acid (Chapter 81), and dicarboxylic acidemias associated with defective fatty acid degradation (Chapter 80.1).
Maple Syrup Urine Disease (MSUD)
Decarboxylation of leucine, isoleucine, and valine is accomplished by a complex enzyme system (branched-chain α-ketoacid dehydrogenase) using thiamine pyrophosphate (vitamin B1) as a coenzyme. This mitochondrial enzyme consists of 4 subunits: E1α, E1β, E2, and E3. The E3 subunit is shared with 2 other dehydrogenases in the body, namely pyruvate dehydrogenase and α-ketoglutarate dehydrogenase. Deficiency of this enzyme system causes MSUD (see Fig. 79-4), named after the sweet odor of maple syrup found in body fluids, especially urine. Based on clinical findings and response to thiamine administration, 5 phenotypes of MSUD have been identified.
Classic MSUD
This form has the most severe clinical manifestations. Affected infants who are normal at birth develop poor feeding and vomiting in the 1st wk of life; lethargy and coma may ensue within a few days. Physical examination reveals hypertonicity and muscular rigidity with severe opisthotonos. Periods of hypertonicity may alternate with bouts of flaccidity. Neurologic findings are often mistaken for generalized sepsis and meningitis. Cerebral edema may be present; convulsions occur in most infants, and hypoglycemia is common. In contrast to most hypoglycemic states, correction of the blood glucose concentration does not improve the clinical condition. Routine laboratory findings are usually unremarkable, except for metabolic acidosis. Death usually occurs in untreated patients in the 1st few weeks or months of life.
Diagnosis is often suspected because of the peculiar odor of maple syrup found in urine, sweat, and cerumen (see Fig. 79-6). It is usually confirmed by amino acid analysis showing marked elevations in plasma levels of leucine, isoleucine, valine, and alloisoleucine (a stereoisomer of isoleucine not normally found in blood) and depression of alanine. Leucine levels are usually higher than those of the other 3 amino acids. Urine contains high levels of leucine, isoleucine, and valine and their respective ketoacids. These ketoacids may be detected qualitatively by adding a few drops of 2,4-dinitrophenylhydrazine reagent (0.1% in 0.1 N HCl) to the urine; a yellow precipitate of 2,4-dinitrophenylhydrazone is formed in a positive test. Neuroimaging during the acute state may show cerebral edema, which is most prominent in the cerebellum, dorsal brainstem, cerebral peduncle, and internal capsule. After recovery from the acute state and with advancing age, hypomyelination and cerebral atrophy may be seen in neuroimaging of the brain. The enzyme activity can be measured in leukocytes and cultured fibroblasts.
Treatment after recovery from the acute state requires a diet low in branched-chain amino acids. Synthetic formulas devoid of leucine, isoleucine, and valine are available commercially. Because these amino acids cannot be synthesized endogenously, small amounts of them should be added to the diet; the amount should be titrated carefully by performing frequent analyses of the plasma amino acids. A clinical condition resembling acrodermatitis enteropathica occurs in affected infants whose plasma isoleucine concentration becomes very low; addition of isoleucine to the diet causes a rapid and complete recovery. Patients with MSUD should remain on the diet for the rest of their lives. Liver transplantation has been performed in a number of patients with classic MSUD with promising results. These children have been able to tolerate a normal diet.
The long-term prognosis of affected children remains guarded. Severe ketoacidosis, cerebral edema, and death may occur during any stressful situation such as infection or surgery, especially in mid-childhood. Mental and neurologic deficits are common sequelae.
Intermittent MSUD
In this form of MSUD, seemingly normal children develop vomiting, odor of maple syrup, ataxia, lethargy, and coma during any stress or catabolic state such as infection or surgery. During these attacks, laboratory findings are indistinguishable from those of the classic form, and death may occur. Treatment of the acute attack of intermittent MSUD is similar to that of the classic form. After recovery, although a normal diet is tolerated, a diet low in branched-chain amino acids is recommended. Activity of dehydrogenase in patients with the intermittent form is higher than in the classic form and may reach 5-20% of the normal activity.
Mild (Intermediate) MSUD
In this form, affected children develop milder disease after the neonatal period. Clinical manifestations are insidious and limited to the CNS. Patients have mild to moderate mental retardation (usually after 5 mo of age) with or without seizures. They have the odor of maple syrup and excrete moderate amounts of the branched-chain amino acids and their ketoacid derivatives in the urine. Plasma concentrations of leucine, isoleucine, and valine are moderately increased whereas those of lactate and pyruvate are normal. These children are commonly diagnosed during an intercurrent illness when signs and symptoms of classic MSUD may occur. The dehydrogenase activity is 3-30% of normal. Because patients with thiamine-responsive MSUD usually have manifestations similar to those seen in the mild form, a trial of thiamine therapy is recommended. Diet therapy, similar to that of classic MSUD, is needed.
Thiamine-Responsive MSUD
Some children with mild or intermediate forms of MSUD who are treated with high doses of thiamine have dramatic clinical and biochemical improvement. Although some respond to treatment with thiamine at 10 mg/24 hr, others may require as much as 200 mg/24 hr for at least 3 wk before a favorable response is observed. These patients also require diets deficient in branched-chain amino acids. The enzymatic activity in these patients is 2-40% of normal.
MSUD Due to a Deficiency of E3 Subunit (Dihydrolipoyl Dehydrogenase)
This is a very rare disorder. Patients develop lactic acidosis in addition to signs and symptoms similar to those of intermediate MSUD because the E3 subunit is also a component of pyruvate dehydrogenase and α-ketoglutarate dehydrogenase. Progressive neurologic impairment manifested by hypotonia and developmental delay occurs after 2 mo of age. Abnormal movements progress to ataxia. Death may occur in early childhood.
Laboratory findings include persistent lactic acidosis with high levels of plasma lactate, pyruvate, and alanine. Plasma concentrations of branched-chain amino acids are moderately increased. Patients excrete large amounts of lactate, pyruvate, α-glutarate, and the 3 branched-chain ketoacids in their urine.
No effective treatment is available. Dietary restriction of branched-chain amino acids and treatment with high doses of thiamine, biotin, and lipoic acid have been ineffective.
Genetics and Prevalence of MSUD
All forms of MSUD are inherited as an autosomal recessive trait. The gene for each subunit resides on different chromosomes. The gene for E1α is on chromosome 19q13.1-q13.2; that for E1β is on chromosome q14; the gene for E2 is on chromosome 1p31; and that for E3 is on chromosome 7q31-q32. Many different disease-causing mutations have been identified in patients with different forms of MSUD. A given phenotype is caused by a variety of genotypes; patients from different pedigrees with the classic form of MSUD have been shown to have mutations in genes for E1α, E1β, or E2. Most patients are compound heterozygotes inheriting 2 different mutant alleles. Mutations in genes for E1β (38%) and E 1α (33%) account for about 70% of cases.
The prevalence is estimated at 1/185,000. The classic form of MSUD is more prevalent in the Old Order Mennonites in the USA, with estimated prevalence of 1/358. Affected patients in this population are homozygous for a specific mutation (Y393N) in the E1α subunit gene.
Early detection of MSUD is feasible by mass screening of newborn infants. Prenatal diagnosis has been accomplished by enzyme assay of the cultured amniocytes, cultured chorionic villi tissue, or direct assay of the chorionic villi samples and by identification of the mutant gene.
Several successful pregnancies have occurred in women with different forms of MSUD. No ill effects have been observed in the offspring of these patients. Episodes of metabolic decompensations have occurred in the mothers during pregnancy and the postpartum period.
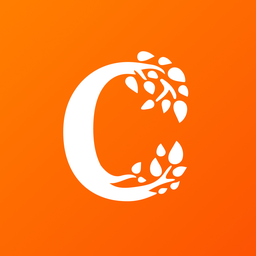
Full access? Get Clinical Tree
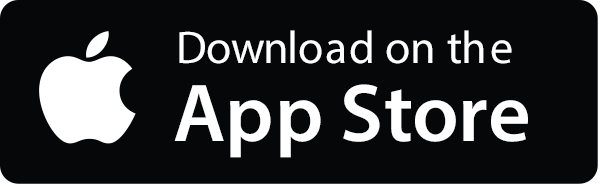
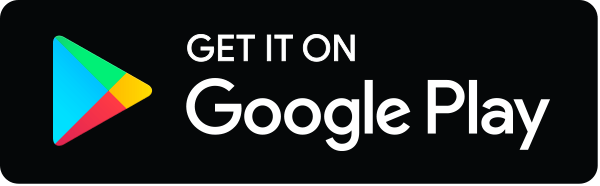