Maternal diabetes-induced birth defects occur in 6-10% of babies born to mothers with pregestational diabetes, representing a significant maternal-fetal health problem. Currently, these congenital malformations represent a significant maternal-fetal medicine issue, but are likely to create an even greater public health threat as 3 million women of reproductive age (19-44 years) have diabetes in the United States alone, and this number is expected to double by 2030. Neural tube defects (NTDs) and congenital heart defects are the most common types of birth defects associated with maternal diabetes. Animal studies have revealed that embryos under hyperglycemic conditions exhibit high levels of oxidative stress resulting from enhanced production of reactive oxygen species and impaired antioxidant capability. Oxidative stress activates a set of proapoptotic kinase signaling intermediates leading to abnormal cell death in the embryonic neural tube, which causes NTD formation. Work in animal models also has revealed that maternal diabetes triggers a series of signaling intermediates: protein kinase C (PKC) isoforms, PKCα, βII and δ; apoptosis signal-regulating kinase 1; c-Jun-N-terminal kinase (JNK)1/2; caspase; and apoptosis. Specifically, maternal diabetes in rodent models activates the proapoptotic unfolded protein response and endoplasmic reticulum (ER) stress. A reciprocal causation between JNK1/2 activation and ER stress exists in diabetic embryopathy. Molecular studies further demonstrate that deletion of the genes for Prkc , Ask1 , Jnk1 , or Jnk2 abolishes maternal diabetes-induced neural progenitor apoptosis and ameliorates NTD formation. Similar preventive effects are also observed when apoptosis signal-regulating kinase 1, JNK1/2, or ER stress is inhibited. Cell membrane stabilizers and antioxidant supplements are also effective in prevention of diabetes-induced birth defects. Mechanistic studies have revealed important insights into our understanding the cause of diabetic embryopathy and have provided a basis for future interventions against birth defects or other pregnancy complications associated with maternal diabetes. The knowledge of a molecular pathway map identified in animal studies has created unique opportunities to identify molecular targets for therapeutic intervention.
Each year in the United States, about 150,000 babies–3% of all live births–are born with at least 1 major congenital malformation. The prevalence of birth defects is significantly worse in offspring of women who have type 1 or 2 diabetes. In these cases, 6-10% of babies are born with a major congenital malformation. Based on the National Health and Nutrition Examination Survey, conducted from 1988 through 1994, 1.1% of women 20-39 years of age have type 1 or 2 diabetes, and the incidence of diabetes among women of childbearing age has increased over the past 4 decades. It is projected that the number of women of childbearing age with type 2 diabetes will double by 2030, suggesting that approximately 8000 babies will be born each year in the United States with a congenital malformation in pregestational type 1 or 2 diabetic pregnancies.
Observational studies in human beings have demonstrated a strong link between the extent of a mother’s glycemic control and the incidence of congenital malformations in her offspring. The putative teratogenic effects of hyperglycemia are supported by studies demonstrating that clinical intervention targeted at achieving euglycemia can reduce the incidence of diabetes-associated birth defects. When euglycemia is successfully maintained periconceptionally and during the first trimester, the prevalence of malformations is reduced to a level comparable to that of the general population. However, even with excellent compliance and clinical care, euglycemia may be difficult to achieve and maintain. In addition, it is possible that organogenesis can be affected by short periods of hyperglycemia that are not reflected in the averaged values of glycosylated hemoglobin levels used to monitor glucose levels. A further obstacle is that most women with diabetes do not seek preconceptional care and most have unplanned pregnancies.
Hence, a very important public health goal is to develop and implement new and easily accessible intervention strategies to decrease the occurrence of diabetes-induced congenital anomalies. To achieve this goal, we need a thorough understanding of the biochemical and molecular mechanisms underlying diabetic embryopathy. Although we are still far from reaching this goal, one area where we have made progress is in our understanding of the link between maternal hyperglycemia and oxidative stress. The molecular pathways involved in the cellular response to stress are potential therapeutic targets to prevent diabetes-induced embryonic malformations.
Excess apoptosis is a causal event in the induction of malformations
Diabetes-associated malformations may involve ≥1 organs and frequently result in significant disability or death. Adverse effects of maternal hyperglycemia have been documented in the yolk sac of diabetic animal models and in cultured murine embryos. Studies with in vivo and in vitro models have determined that the stages of embryogenesis vulnerable to hyperglycemia-induced malformations comprise the critical period of organogenesis between 8.5-11.5 and 9-12 days of gestation in the mouse and rat, respectively, which is equivalent to gestational weeks 3-5 in human beings.
Both clinical cases and animal studies have clearly demonstrated that the main characteristics of maternal hyperglycemia-associated defects are organ agenesis and underdevelopment. The organ systems most commonly affected include the central nervous, cardiovascular, gastrointestinal, craniofacial, genitourinary, and skeletal systems. Because the neural folds and the heart develop early during embryogenesis, a higher incidence of malformations is observed in these organs. In the central nervous system, abnormalities can be categorized as underdevelopment of the midbrain and hindbrain, and failure of the neural tube to close at both anterior (rostral) and posterior (caudal) ends of the neural axis. The failure of posterior neural tube closure results in spina bifida, 1 of the common birth defects among offspring of diabetic mothers.
Multiple studies have confirmed that excessive cell death, at least in the central nervous system, contributes to the abnormal development of structures in the embryos of diabetic animals. These observations strongly suggest that high concentrations of glucose cause damage to the neural progenitor cells, leading to apoptosis and, ultimately, abnormal organogenesis. However, the mechanisms by which hyperglycemia triggers cell death in the embryonic cells are largely unknown.
Programmed cell death is a precisely controlled cellular event that can be triggered by extracellular signals or other stimuli under normal and pathological conditions. In most cases, apoptosis is characterized by the condensation of chromatin, degradation (fragmentation) of DNA, and formation of apoptotic bodies. The intracellular factors activated during apoptosis are the members of the Bcl-2 family, notably Bax and Bim. When apoptosis initiates, Bax and Bim become activated. Activated Bax moves to the mitochondrion to form a transmembrane channel with Bak, another Bcl-2 family member. Bim is phosphorylated and translocates to the mitochondria to help open the Bax/Bak channel, resulting in cytochrome C release into the cytosol. Cytochrome C binds to apoptosis protease-activating factor-1, and the resulting complex activates caspase-9. Activated caspase-9 activates caspase-3, which then turns on caspase-activated DNase and other proapoptotic factors, leading to DNA fragmentation and cell death.
Hyperglycemia-induced oxidative stress
Evidence from clinical and experimental studies demonstrates that diabetes-related hyperglycemia leads to sustained generation of reactive oxygen species (ROS) and depletion of antioxidants, resulting in intracellular oxidative stress from an imbalance in intracellular reduction-oxidation (redox) homeostasis. Under normal physiological conditions, oxygen-free radicals, including hydroxyl radicals, superoxide anions, singlet oxygen, and hydrogen peroxide, are produced during cellular energy metabolism in mitochondria. Physiologic levels of ROS mediate intracellular signal transduction, which, in turn, regulates a wide range of cellular functions, including proliferation, differentiation, and migration. However, under pathological conditions, excess ROS can oxidize proteins, lipids, and DNA, causing cell injury and cell death ( Figure 1 ).
Intracellular redox homeostasis depends on the relative balance between ROS production and the thiol buffers, glutathione (GSH) and thioredoxin. Normally, the intracellular environment is maintained in a highly reduced state, which is mediated by high levels of reduced GSH and thioredoxin. However, ROS produced via various cellular activities converts GSH into oxidized GSH disulfide ( Figure 1 ). If ROS production exceeds the cellular thiol-buffering capacity, the oxidizing agents build up in the cell, causing damage and promoting oxidative stress.
In addition to the GSH antioxidant buffering system, cells also protect themselves by producing antioxidative enzymes that convert damaging radicals to nontoxic molecules ( Figure 1 ). Superoxide dismutases (SODs) convert a superoxide anion into hydrogen peroxide, which is then reduced to water by GSH peroxidase and catalase. Two types of mammalian intracellular SODs, copper-zinc SOD (SOD1) and manganese SOD (SOD2), have been extensively studied ; SOD1 is localized in the cytoplasm, SOD2 in the mitochondrial matrix. It has been shown that SOD1 not only controls the redox state in the cytoplasm, but also regulates mitochondrial homeostasis. A study using transgenic mouse embryos overexpressing a human SOD1 transgene showed higher SOD activity and lower malformation rate in response to maternal diabetic conditions than wild-type embryos under the same conditions. These studies strongly suggest that antioxidative enzymes play an important role in protecting embryos against hyperglycemia-augmented oxidative stress ( Figure 1 ).
The effects of ROS can be transduced by a number of factors within the cell, including p66Shc, a member of the ShcA family which also includes p46Shc and p52Shc ( Figure 1 ). Unlike other members of this protein family, p66Shc is a specific target of ROS and a critical transducer of oxidative stress signaling leading to apoptosis. p66Shc is activated via phosphorylation of its serine 36 residue in the CH2 domain. Targeted deletion of the p66Shc gene in the mouse increases cellular resistance to oxidative stress-induced apoptosis. The apoptotic effect of p66Shc may involve functional activation and/or transcriptional regulation of Bax and Bim ( Figure 1 ). Recently, reports in animal models have shown that p66Shc plays a critical role in diabetic complications. In our laboratory, p66Shc is also affected in models of diabetic embryopathy. These observations suggest that p66Shc may be an important factor that mediates the effects of oxidative stress in diabetes-induced birth defects.
Hyperglycemia-induced oxidative stress
Evidence from clinical and experimental studies demonstrates that diabetes-related hyperglycemia leads to sustained generation of reactive oxygen species (ROS) and depletion of antioxidants, resulting in intracellular oxidative stress from an imbalance in intracellular reduction-oxidation (redox) homeostasis. Under normal physiological conditions, oxygen-free radicals, including hydroxyl radicals, superoxide anions, singlet oxygen, and hydrogen peroxide, are produced during cellular energy metabolism in mitochondria. Physiologic levels of ROS mediate intracellular signal transduction, which, in turn, regulates a wide range of cellular functions, including proliferation, differentiation, and migration. However, under pathological conditions, excess ROS can oxidize proteins, lipids, and DNA, causing cell injury and cell death ( Figure 1 ).
Intracellular redox homeostasis depends on the relative balance between ROS production and the thiol buffers, glutathione (GSH) and thioredoxin. Normally, the intracellular environment is maintained in a highly reduced state, which is mediated by high levels of reduced GSH and thioredoxin. However, ROS produced via various cellular activities converts GSH into oxidized GSH disulfide ( Figure 1 ). If ROS production exceeds the cellular thiol-buffering capacity, the oxidizing agents build up in the cell, causing damage and promoting oxidative stress.
In addition to the GSH antioxidant buffering system, cells also protect themselves by producing antioxidative enzymes that convert damaging radicals to nontoxic molecules ( Figure 1 ). Superoxide dismutases (SODs) convert a superoxide anion into hydrogen peroxide, which is then reduced to water by GSH peroxidase and catalase. Two types of mammalian intracellular SODs, copper-zinc SOD (SOD1) and manganese SOD (SOD2), have been extensively studied ; SOD1 is localized in the cytoplasm, SOD2 in the mitochondrial matrix. It has been shown that SOD1 not only controls the redox state in the cytoplasm, but also regulates mitochondrial homeostasis. A study using transgenic mouse embryos overexpressing a human SOD1 transgene showed higher SOD activity and lower malformation rate in response to maternal diabetic conditions than wild-type embryos under the same conditions. These studies strongly suggest that antioxidative enzymes play an important role in protecting embryos against hyperglycemia-augmented oxidative stress ( Figure 1 ).
The effects of ROS can be transduced by a number of factors within the cell, including p66Shc, a member of the ShcA family which also includes p46Shc and p52Shc ( Figure 1 ). Unlike other members of this protein family, p66Shc is a specific target of ROS and a critical transducer of oxidative stress signaling leading to apoptosis. p66Shc is activated via phosphorylation of its serine 36 residue in the CH2 domain. Targeted deletion of the p66Shc gene in the mouse increases cellular resistance to oxidative stress-induced apoptosis. The apoptotic effect of p66Shc may involve functional activation and/or transcriptional regulation of Bax and Bim ( Figure 1 ). Recently, reports in animal models have shown that p66Shc plays a critical role in diabetic complications. In our laboratory, p66Shc is also affected in models of diabetic embryopathy. These observations suggest that p66Shc may be an important factor that mediates the effects of oxidative stress in diabetes-induced birth defects.
Oxidative stress-induced proapoptotic protein kinase C signaling
Intracellular ROS are generated by a number of mechanisms, including changes in ion homeostasis, membrane lipid metabolism, and peroxidation. In embryos under maternal hyperglycemic conditions, products of arachidonic acid (AA) metabolism (lipoperoxides) have been detected. The major pathway of AA metabolism involves cyclooxygenase-2-catalyzed production of prostaglandin E 2 (PGE 2 ). Adding PGE 2 to the medium of embryos cultured under high-glucose conditions prevents malformations, suggesting that PGE 2 has a protective effect on embryos exposed to hyperglycemia.
Other pathways of AA metabolism have also been identified in diabetic patients. In these alternate pathways, AA is converted into PGE 2 -like isoprostanes, such as 8-iso-prostagladin F 2 (PGF 2 ) and 8-iso-PGF 2 α, by noncyclooxygenase-catalyzed peroxidation involving free radicals. These PGE 2 -like isoprostanes have been shown to have damaging effects in animal models and embryos. In embryos cultured under hyperglycemic conditions, as well as in diabetic patients, the level of 8-iso-PGF 2 is dramatically elevated, suggesting a shift in metabolism from AA/PGE 2 to AA/isoprostanes ( Figure 2 ).
Dietary AA appears to be protective against hyperglycemia-induced damage. We have shown that pregnant diabetic rats supplemented with AA display a reduced incidence of embryonic malformations. Similar phenomena have also been seen with the addition of AA to embryos cultured in high concentrations of glucose. Giving AA as a treatment may protect against hyperglycemic insults because exogenous AA may replace the endogenous AA displaced from the cell, thereby repairing and stabilizing cell membrane structure and function.
The protein kinase C (PKC) family of serine/threonine protein kinases consists of 12 members, which can be divided into the following 3 groups based on their activation mechanisms : (1) PKCα, β1, β2, and γ require calcium and diacylglycerol (DAG) for activation; (2) PKCδ, ε, η, ν, and θ require only DAG; and (3) PKCμ, ξ, and ι/λ do not require calcium or DAG, but instead require distinct lipid cofactors (eg, ceramide and phosphatidylinositol-4-phosphate). Substrate specificity of an individual PKC family member involves binding to its specific membrane-bound anchor protein and becoming localized to a particular cellular compartment, such as the plasma membrane, cytoskeleton, mitochondrion, or nucleus. PKCs are involved in a number of cellular activities, including proliferation, migration, apoptosis, differentiation, and secretion.
Prolonged activation of PKC by hyperglycemia has been documented in people with diabetes, animal models, and cultured cells. Specific PKC isoforms (α, β2, and δ) are up-regulated, while others (ε and ξ) are down-regulated in diabetic embryopathy ( Figure 2 ). Pharmacological inhibition of the activity of PKCα, -β2, and -δ results in significant decreases in neural tube defect (NTD) rates in embryos cultured under high-glucose conditions. Molecular studies have further uncovered a functional role for PKCβII activation in diabetic embryopathy.
Evidence suggests that maternal diabetes-induced oxidative stress is a major contributor to PKC activation. Transgenic overexpression of SOD1, which suppresses oxidative stress and NTD formation represses maternal diabetes-induced phosphorylation of PKCα/βII and PKCδ. In addition, PKC activation induces lipid peroxidation ( Figure 2 ) and, thus, may further enhance the degree of oxidative stress seen in embryos subjected to hyperglycemic conditions. Therefore, our experiments have shown that oxidative stress and PKC activation form a positive feedback loop in diabetic embryopathy ( Figure 2 ).
Hyperglycemia and oxidative stress-altered mitogen-activated protein kinase signaling
Chronic and excessive oxidative stress results in cell injury and activation of a variety of stress-sensitive signaling pathways that often induce apoptosis. Members of the mitogen-activated protein kinase (MAPK) family play a large role in programmed cell death and are activated in response to a variety of extracellular stimuli, including ROS. Activation of these serine/threonine kinases requires phosphorylation. MAPKs can be grouped into extracellular signal-regulated kinases (ERKs), c-jun N-terminal kinases (JNKs)/stress-activated protein kinases, p38, and others ( Figure 3 ). MAPK activity is altered in diabetic patients and in cells cultured in high glucose, suggesting that MAPKs may be involved in hyperglycemia-induced complications. Although MAPKs are involved in various cellular activities, the ERK pathways primarily mediate cell proliferation, and the JNK and p38 pathways respond to cell stress signals and mediate apoptosis. Maternal diabetes induces JNK1/2 activation but suppresses ERK phosphorylation associated with increased apoptosis in the developing embryo ( Figure 3 ).
Three members of the JNK family, JNK1, -2, and -3, and their splice variants have been characterized. JNK-1 and JNK-2 are ubiquitously expressed, while JNK3 is primarily expressed in the nervous system. JNKs are activated by phosphorylation by upstream MAPK kinases (MKKs), specifically MKK4 and MKK7. MKKs are, in turn, phosphorylated by other enzymes, one of which is apoptosis signal-regulating kinase 1 (ASK1), a kinase that is activated under the influence of ROS ( Figure 3 ). JNKs can phosphorylate nuclear proteins, such as c-jun, ATF2, and Elk-1, as well as cytoplasmic proteins, such as Bcl-2 and Bim. Mice with a null mutation in any individual jnk gene develop normally, as do double mutants of jnk1 / jnk3 or jnk2 / jnk3 . Although jnk1 / jnk2 null mutants die in utero due to an abundance of abnormal apoptosis in the brain, individual jnk gene null mutants are still useful models for delineating apoptotic pathways involving JNKs. It remains unclear how JNK1/2 are activated by maternal diabetes. It is likely that JNK1/2 are activated by oxidative stress because overexpressing the antioxidant enzyme SOD1 in transgenic mice abrogates maternal diabetes-induced JNK1/2 activation.
Recently, we have revealed a functional role for JNK1/2 activation in diabetic embryopathy. In a cultured embryo system, inhibiting JNK1/2 by the pharmacological inhibitor, SP600125, reduced high glucose-induced NTD formation, whereas adding sorbitol, a JNK1/2 activator, induced NTD formation. Studies using gene knockout mouse models have uncovered a critical role for JNK1/2 activation in maternal diabetes-induced apoptosis and NTD formation. Deletion of either the Jnk1 gene or the Jnk2 gene abolishes the activation of 4 transcription factors downstream of JNK1/2, and blocks maternal diabetes-induced caspase cascade activation, neural progenitor apoptosis, and NTD formation. These findings support the hypothesis that JNK1 and JNK2 are equally responsible for the induction of diabetic embryopathy, and that the JNK1/2 pathway mediates apoptosis and the teratogenicity of maternal diabetes ( Figure 3 ).
The reciprocal causation between JNK1/2 activation and endoplasmic reticulum stress
JNK1/2 activation induces proapoptotic cellular events leading to apoptosis. JNK1/2 activation positively modulates the activities and mitochondrial translocation of the proapoptotic Bcl-2 family members ( Figure 3 ). While both JNK1/2 and increased activities of proapoptotic Bcl-2 family members have been observed in diabetic embryopathy, the relationship between these events has not been established in relation to mitochondrial dysfunction, which is manifested in embryos exposed to maternal diabetes. Recently, endoplasmic reticulum (ER) stress has emerged as a proapoptotic event that is involved in the pathogeneses of diabetic complications. It is known that 1 of the unfolded protein response (UPR) sensors, inositol-requiring protein (IRE)-1α, can activate JNK1/2 under ER stress conditions ( Figure 4 ). In our previous ultracellular study using electronic microscopy, aberrant maturational and cytoarchitectural changes associated with malformations in cultured embryos were observed under high-glucose conditions. These findings suggest that ER stress may be present in embryos exposed to maternal diabetes, and plays a role in JNK1/2 activation and apoptosis.
Newly synthesized proteins are folded into their correct 3-dimensional structures in the ER. A group of molecular chaperone proteins residing in the ER, such as binding immunoglobulin protein and calnexin, is critical for the maintenance of ER luminal homeostasis. Accumulation of misfolded proteins due to ER luminal imbalance triggers ER stress and the induction of apoptosis. The UPR sensors, particularly IRE1α and protein kinase RNA-like endoplasmic reticulum kinase (PERK), mediate proapoptotic signaling in the ER ( Figure 4 ).
Our recent study demonstrated that neuroepithelial cells in the developing neural tubes of embryos exposed to maternal diabetic conditions possess swollen/stressed ER lumens, and have elevated ER stress markers. Our work in animal models has shown that maternal diabetes activates IRE1α and PERK. IRE1α–activation leads to splicing of X-box binding protein messenger RNA and subsequent formation of a transcription activator, whereas PERK activation results in phosphorylation of eukaryotic initiation factor 2α leading to up-regulation of an apoptotic factor, C/EBP-homologous protein ( Figure 4 ).
Our laboratory has further revealed a causal role for ER stress in maternal diabetes-induced neuroepithelial cell apoptosis and NTD formation by blocking ER stress. We have shown that the ER stress inhibitor, 4-phenylbutyric acid (PBA), diminishes ER stress markers, and blocks apoptosis in the developing neural tube and NTD formation in embryos cultured under high-glucose conditions ( Figure 4 ). Because it is widely accepted that ER stress activates JNK1/2, we have conducted subsequent studies to show that 4-PBA treatment also can suppress hyperglycemia-induced JNK1/2 activation. The relationship between JNK1/2 activation and ER stress has been further defined using JNK1 and JNK2 knockout mice. Deletion of either Jnk1 or Jnk2 gene abolishes maternal diabetes-triggered UPR signaling and ER stress, indicating that JNK1/2 activation acts upstream of ER stress ( Figure 4 ). The observations we have made in our diabetic embryopathy model system agree with findings in another recent study, which showed that inhibiting JNK1/2 prevented ER stress and apoptosis in pancreatic cells. Therefore, our work has indicated a reciprocal causation between JNK1/2 and ER stress exists in pathogenesis of diabetic embryopathy ( Figure 4 ).
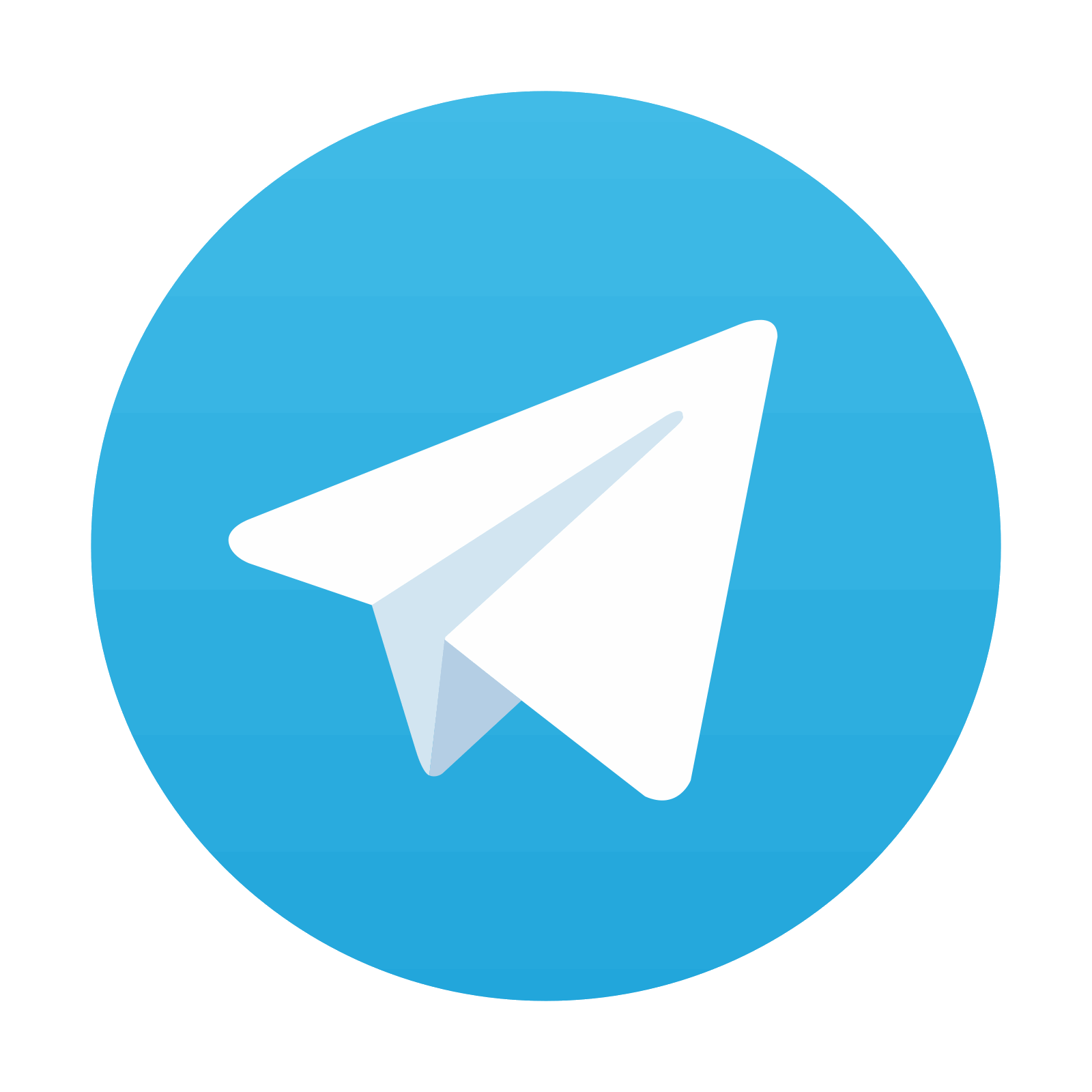
Stay updated, free articles. Join our Telegram channel
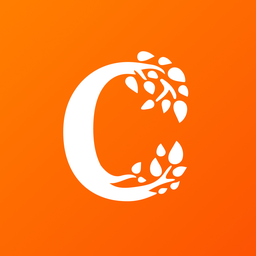
Full access? Get Clinical Tree
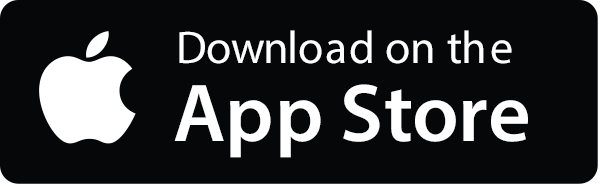
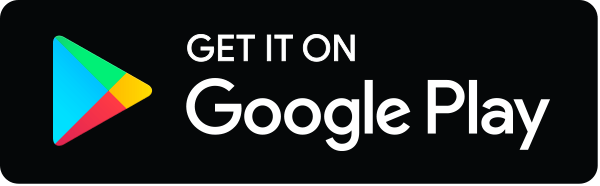