Clinical Pharmacology of Antineoplastic Drugs
Stacey L. Berg
Lisa R. Bomgaars
David G. Poplack
Challenges inherent in administering antineoplastic therapy safely to children are numerous. Among the most critical issues are the toxicity of antineoplastic drugs and the developmental heterogeneity of the pediatric population. Antineoplastic agents have the lowest therapeutic index of any drugs in clinical use. Because cancer is life-threatening, clinicians and patients are willing to tolerate toxicity from anticancer agents that would be intolerable for any other class of drugs. Furthermore, these agents are virtually always used in combination, even when the interactions among the agents selected are poorly understood. Children of any age, from newborn to adolescent, may require therapy, yet the impact of developmental factors on the pharmacology of antineoplastic drugs is seldom known. In particular, neonates and young infants, who are most likely to differ physiologically from older children and adults, represent such a small number of pediatric cancer cases that there is relatively little opportunity to study the clinical pharmacology of drugs in this population, even though it may be most vulnerable to ignorance.
In this chapter, we apply general principles of pharmacology to an overview of the antineoplastic drugs commonly used to treat childhood cancer. The approach to understanding drugs by studying their absorption, distribution, metabolism, and elimination can be applied to the antineoplastic agents. However, because these agents do not comprise one homogeneous class, it is helpful also to consider mechanism of action as a general characteristic that must be understood about each drug before the pharmacology of the agent can be fully appreciated. In this chapter, we illustrate the way that each basic principle may be applied to antineoplastic agents using specific drugs as examples, then discuss the way that understanding individual drugs can be used to design the combination chemotherapy regimens that are the cornerstone of anticancer treatment for children. Finally, we review special considerations in the pharmacology of antineoplastic drugs in infants and younger children.
Mechanisms of Action
Understanding drug mechanism of action is useful in making rational decisions about the dose and administration schedule for individual drugs as well as about logical ways to combine drugs into multiagent regimens. For example, antimetabolites are considered cell cycle phase specific because they produce cytotoxicity during S phase, when they can be incorporated as fraudulent substrates into replicating DNA. Thus, these agents are often administered by prolonged infusion or in multiple daily doses to increase the likelihood that drug will be present when cells are passing through S phase. Similarly, logical combinations of agents may be based on nonoverlapping mechanisms of resistance or nonoverlapping toxicities, but they may also take advantage of mechanism of action by inhibiting the same intracellular process at different points in its pathway or by taking advantage of lesions induced by one drug to potentiate the activity of another (as discussed later) (1). Some commonly used anticancer drugs and their mechanisms of action are listed in Table 44.1.
Many anticancer drugs work by interfering at some stage with the synthesis or function of DNA and RNA in tumor cells. These disruptions usually induce apoptosis as the final common pathway of cell death and are not tumor specific (2,3,4,5). In the past, potential anticancer drugs were usually identified in general screens for cytotoxicity, such as the National Cancer Institute panel of tumor cell lines (6,7). More recently, however, advances in understanding tumor biology, especially at the molecular level, have led to the rational development of agents that act more specifically on abnormal molecules or pathways within tumor cells. These targeted agents may theoretically be both more active against tumors and less toxic to normal tissues. The enthusiasm generated by this approach makes it likely that the proportion of molecularly targeted new drugs will increase and that there will be less emphasis on the development of nonspecific cytotoxic agents in the future (8,9). However, the ability to apply these new targeted agents properly will depend on detailed understanding of the drug’s targeted pathway in the pathogenesis of pediatric malignancies (10).
Table 44.1 Mechanism of Action of Commonly Used Anticancer Drugs | |||||||||||||||||||||||||||||||||||||||||||||||||||||||||||||||||||||
---|---|---|---|---|---|---|---|---|---|---|---|---|---|---|---|---|---|---|---|---|---|---|---|---|---|---|---|---|---|---|---|---|---|---|---|---|---|---|---|---|---|---|---|---|---|---|---|---|---|---|---|---|---|---|---|---|---|---|---|---|---|---|---|---|---|---|---|---|---|
|
Many conventional anticancer agents exert their cytotoxicity by producing inter- and intrastrand cross-links or strand breaks in DNA. Alkylating agents such as nitrogen mustard, cyclophosphamide, ifosfamide, melphalan, and dacarbazine form covalent bonds with intracellular molecules, including DNA as the primary target. The bifunctional alkylating agents, which include nitrogen mustard, the oxazaphosphorines cyclophosphamide and ifosfamide, and melphalan, have two reactive groups that can form intra- and interstrand cross-links that inactivate DNA (11). Although cisplatin and carboplatin are not classical alkylating agents, they also undergo similar chemical reactions to form covalent bonds and cross-links like those formed by alkylating agents (12).
The anthracycline antitumor antibiotics doxorubicin, daunomycin, and idarubicin and the related compound actinomycin D have planar multiring structures that can intercalate into the double helix of DNA, interfering with its replication and transcription. The cytotoxicity of the anthracyclines results from DNA strand breaks that are mediated by the enzyme topoisomerase-II; the exact mechanism of action is not fully explicated (13). The anthracyclines also undergo enzymatic reduction to form free radicals that undergo further reactions to produce to hydrogen peroxide and hydroxyl radicals. It is not known whether these highly reactive compounds contribute to the antitumor effect of the anthracyclines, but they may contribute in part to their well-known cardiac toxicity (13,14,15). The principal metabolites of the anthracyclines are the corresponding alcohols, doxorubicinol, daunomycinol, and idarubicinol, formed by the action of aldoketoreductase (see below).
The epipodophyllotoxins etoposide (VP-16) and teniposide (VM-26) also interact with topoisomerase II to produce DNA strand breaks, but they do not intercalate into DNA. Topoisomerase-II is normally responsible for opening and rejoining DNA during replication, but in the presence of an epipodophyllotoxin, the strand-closing reaction is blocked (16,17).
The antimetabolites, structural analogues of normal intracellular molecules, act as fraudulent substrate enzymes in critical biochemical pathways. Antimetabolites are among the oldest anticancer agents in clinical use, and they can be viewed as rationally synthesized agents because they are specifically designed to take advantage of well-characterized biochemical pathways, although the pathways are not specific to tumor cells. Antimetabolites exert their cytotoxicity by inhibiting enzymatically catalyzed steps in the synthesis of nucleic acids or by being incorporated into DNA or RNA, resulting in defective products. The antimetabolites most commonly used in children include cytarabine (ara-C), 6-mercaptopurine (MP), thioguanine (TG), and methotrexate. Newer antimetabolites include nelarabine (Ara-G) (18,19,20) gemcitabine (21,22,23), capecitabine (24,25), and clofarabine (26). While the role of gemcitabine and capecitabine in pediatric cancer treatment is not yet established, nelarabine is active in pediatric patients with refractory T-cell malignancies (27) and approved for use in that setting, and clofarabine is active and approved for use in patients with relapsed or refractory acute lymphoblastic leukemia (28). The role of these agents in frontline therapy combinations has not yet been defined. Many antimetabolites act as prodrugs that require metabolic activation; this feature will be discussed in detail in the section on biotransformation.
Tubulin, a structural protein that polymerizes to form microtubules, which are critical for successful completion of mitosis, is the intracellular target for several classes of anticancer agents. The Vinca alkaloids vincristine and vinblastine bind to tubulin, thus blocking microtubule assembly, preventing the formation of the mitotic spindle, and inhibiting mitosis (16). In contrast, the taxanes paclitaxel and its semisynthetic analogue docetaxel also interact with microtubules, but these agents block mitosis by stabilizing microtubules, preventing their normal disassembly (29,30,31). Although taxanes have well-defined roles in the treatment of adult cancers, they appear to have limited utility in pediatric tumors and are not widely used.
The bacterial enzyme L-asparaginase has a mechanism of action unique among anticancer agents. This drug, first identified in the 1950s in guinea pig serum (32), depletes the circulating pool of the amino acid L-asparagine, which is nonessential in mammals. Lymphoblasts, however, lack the enzyme that converts aspartic acid to asparagine and therefore are dependent on the presence of L-asparagine to maintain protein synthesis (33). L-asparaginase therapy is an important component of lymphoblastic leukemia treatment. L-asparaginase is commonly administered as either the “native” E. coli-produced product which requires frequent (three times a week) administration or a pegylated product with a longer half-life (34,35).
The camptothecin analogues topotecan and irinotecan are agents that exert their antitumor effect by inhibiting topoisomerase-I, a nuclear enzyme involved in DNA uncoiling during replication (36). Treatment with camptothecins leads to formation of DNA–topoisomerase-I adducts and single-strand DNA breaks (36).
Many new molecular-targeted agents are in development, although for the most part their efficacy in pediatric anticancer therapy is not yet determined. One agent that clearly does have a role is the tyrosine kinase inhibitor imatinib mesylate (Gleevec). This agent inhibits the bcr–abl tyrosine kinase that results from the Philadelphia (9,22) chromosome translocation in chronic myelogenous leukemia (37,38). It also inhibits platelet-derived growth factor receptor (PDGF-R), stem cell factor receptor, and c-kit-mediated signaling (39,40,41). Imatinib mesylate is active in most patients with chronic myelogenous leukemia as well as in some patients with Philadelphia chromosome–positive acute lymphocytic leukemia (ALL) (38,42,43,44,45,46,47,48,49). A number of other molecularly targeted agents also have been approved for use in adult malignancies. A particularly compelling target is the vascular endothelial growth factor pathway (50); bevacizumab (Avastin) is the prototype in this class and is used in combination with chemotherapy in adult lung and colon cancer (51,52). Bevacizumab is actively being studied in a range of childhood cancers. Erlotinib (Tarceva) targets the epidermal growth factor receptor tyrosine kinase (53) and is also being studied in children.
Pharmacokinetics
Pharmacokinetics is the study of drug absorption, distribution, metabolism, and excretion. Understanding these processes may help determine the proper dose, schedule, and route of administration for anticancer agents. In addition, modeling the pharmacokinetic behavior of a drug may yield important insights into relationships among things like dose and toxicity and response. In children, this understanding is particularly important because developmental physiologic differences between children and adults, such as degree of drug absorption, plasma protein or tissue binding, distribution of drug in the various tissues of the body, and excretory organ function, may mean that the appropriate dose and schedule of administration of a given drug might be quite different in children from adults. In addition, understanding drug elimination pathways can be helpful when recommending dose adjustments for patients with hepatic or renal dysfunction (54).
Among the most important pharmacokinetic concepts is systemic drug exposure, quantified by determining the area under the plasma concentration–time curve, or AUC. Measuring systemic drug exposure can be labor intensive and often involves collaborative research efforts. For drugs administered by prolonged continuous infusion, determination of the steady-state plasma drug concentration is adequate. However, for drugs administered intermittently, determination of the AUC generally requires sampling at multiple points over a prolonged period. However, detailed pharmacokinetic modeling may permit development of a limited sampling strategy in which the AUC can be predicted from a small number of pharmacokinetic samples after drug distribution is thoroughly described in a relatively small initial group of patients (55,56,57). In addition, parameters other than AUC should also be evaluated for clinical correlations. For example, methotrexate toxicity depends more on the time that drug concentrations stay above certain levels than on the AUC (as discussed later).
Absorption
Absorption refers to the movement of an agent from a peripheral site into the systemic circulation. Because oral administration is the most important nonintravenous route of anticancer drug administration, it is important to consider limitations produced by the gastrointestinal tract. After oral administration, drugs may be degraded by acids in the stomach, adsorbed by food or other medications, metabolized by enzymes in gut luminal cells, extracted by the liver in first-pass metabolism before a systemic effect can be produced, or simply not absorbed because of physicochemical characteristics of the drug molecule. The rate and extent of drug absorption after oral compared with intravenous administration is referred to as bioavailability. Before a drug can be incorporated into anticancer treatment regimen, its bioavailability must be understood. The most reliable way to study bioavailability is to compare plasma AUCs after oral and intravenous administration in the same patient; bioavailability is usually expressed as the fraction of the AUC after intravenous administration produced by oral administration.
The two agents most commonly administered by mouth in childhood anticancer therapy are methotrexate and mercaptopurine (MP), which are used in nearly all maintenance regimens for ALL. Both these agents exhibit considerable variation in bioavailability (58,59,60,61,62,63). Concomitant food administration diminishes the absorption of both agents (64,65). There may also be diurnal variation in the absorption or elimination of these drugs, as suggested by the observation that children who take oral methotrexate and MP in the evening appear to have lower risk of relapse than those who take them in the morning (66,67,68,69).
Bioavailability of methotrexate at low doses (7.5 to 40 mg per m2) is quite variable. In one study, absorption ranged from 23% to 95% (58). Furthermore, methotrexate absorption appears to be saturable, with a plateau in AUC observed at doses of 30 mg per m2 (58,70,71). Therefore, the relationship between dose and AUC is not linear for oral methotrexate. To overcome these limitations, intramuscular administration is sometimes used instead of oral administration of methotrexate. In one study of children with ALL, intramuscular injection of methotrexate resulted in approximately twice the bioavailability of oral administration, and at doses exceeding 40 mg per m2 intramuscular absorption did not show the same nonlinearity as oral administration (71).
Oral administration of MP also results in variable systemic exposure. MP undergoes extensive first-pass metabolism in the liver and gut mucosa by the enzyme xanthine oxidase (63,72). In addition, there is considerable interpatient variability of plasma MP concentrations even when the dose is administered under fasting conditions (61,62,63). Intrapatient variability has also been observed with repeated monitoring of individual patients (73).
Other agents may have more predictable bioavailability after oral administration. The absorption of prednisone and dexamethasone is greater than 80% (74,75), although variability in the absorption of prednisone has been reported in children (76). Oral busulfan is rapidly absorbed, with a bioavailability of 70% in children (77,78). Interestingly, there appears to be circadian variation in busulfan plasma concentrations, with the highest troughs occurring in the early morning (79).
Distribution
Distribution of a drug refers to its transfer among various tissues, blood, tumor, and any other compartments. Although it is possible to measure drug concentrations in different tissues under laboratory conditions, it is not feasible to do so in most clinical settings. Drug concentrations in various compartments can be estimated using pharmacokinetic modeling techniques, but the compartments referred to in models do not usually correlate directly with physiologic compartments. However, the use of pharmacokinetic models, in addition to preclinical studies, permits some conclusions to be drawn about the distribution of most anticancer agents. For example, the pharmacokinetic behavior of both the anthracyclines and the Vinca alkaloids is consistent with extensive tissue binding. Disappearance of these drugs from plasma drug during the distributive phase is extremely rapid, with a distribution half-life of less than 10 minutes. These agents also exhibit volumes of distribution that are much larger than the circulatory volume (several hundred L per m2) as well as a prolonged terminal half-lives (80,81). In addition, tissue levels of anthracyclines, which bind extensively to DNA, are 10- to 500-fold higher than plasma concentrations of the drugs (13).
Methotrexate distributes widely in total body water (82). This is important in relationship to drug toxicity because methotrexate distributes freely into extravascular fluid collections such as pleural effusions or ascites. These fluid collections can contain substantial amounts of methotrexate after high-dose administration and can act as depots that release drug slowly back to other issues, resulting in prolonged systemic drug exposure and increased toxicity (83). Thus, methotrexate should be administered with caution to patients with ascites or pleural effusions, and consideration should be given to draining such fluid collections prior to methotrexate therapy.
Distribution of drugs into the central nervous system (CNS) represents a special challenge in anticancer therapy. Both primary and metastatic CNS tumors are very common in children, and drugs must cross the blood–brain barrier (BBB) to gain access to these tumors. In many tumors the BBB is at least partially disrupted by the tumor itself (84). In other cases, the BBB is relatively intact. Drugs that are poorly soluble in lipids, undergo significant ionization in plasma, or are highly protein bound are unlikely to penetrate extensively across the BBB (85,86,87). Because it is very difficult to measure drug penetration into tumor or brain tissue, the concentration of drug in the cerebrospinal fluid (CSF) is often used as a surrogate marker for CNS penetration. CNS drug penetration is most accurately described expressed as the ratio of drug AUC in CSF to drug AUC in plasma. The majority of anticancer drugs in clinical use do not cross the intact BBB to any significant extent (Table 44.2). CSF:plasma ratios for the Vinca alkaloids, anthracyclines, and epipodophyllotoxins are less than 10%. A few agents, such as thiotepa and the nitrosoureas, penetrate well. Several useful agents, such as cytarabine and methotrexate, do not penetrate well but can be administered in such high systemic doses that even though the CSF:plasma ratio is low, the CSF concentration is still cytotoxic (88,89,90,91,92,93,94). However, such high-dose systemic approaches produce significant systemic toxicity. The most common approach to overcome this problem is the direct intrathecal administration of anticancer agents. Methotrexate and cytarabine remain the mainstay of intrathecal therapy, although other agents, such as thiotepa (95), DepoCyt (liposomally encapsulated cytarabine) (96,97,98,99), topotecan (100), gemcitabine (101,102), and busulfan (103) have also been studied.
Table 44.2 Central Nervous System Penetration of Common Anticancer Agents | ||||||||||||||||||||||||||||||||||||||||||||||||||
---|---|---|---|---|---|---|---|---|---|---|---|---|---|---|---|---|---|---|---|---|---|---|---|---|---|---|---|---|---|---|---|---|---|---|---|---|---|---|---|---|---|---|---|---|---|---|---|---|---|---|
|
Biotransformation
Biotransformation, or metabolism, of anticancer drugs can result in production of active agents or inactivation of the agents. Some commonly administered anticancer agents are really prodrugs that require metabolic transformation before they have antitumor activity (Table 44.3). Other drugs are active in the form administered but undergo metabolism to additional active species that may contribute importantly to activity or toxicity. For these agents, all active moieties must be considered in evaluating the relationship between pharmacokinetic parameters like AUC or half-life and pharmacodynamic parameters like response or toxicity. Drug activation most commonly occurs in the liver, where the bulk of drug metabolism takes place. Some drugs, especially antimetabolites, may be activated in their target tissues as they are incorporated into DNA, RNA, or other macromolecules. A few drugs, such as alkylating agents, undergo spontaneous chemical decomposition in solution to cytotoxic reactive intermediates. Interpatient variability in metabolic activation may be an important part of interpatient variability in drug activity or especially toxicity at a given dose. Saturation of drug-metabolizing enzymes at high dose levels may lead to nonlinear dose-response curves. Interaction or competition with other drugs for a metabolic pathway, or even a drug’s induction of its own metabolic pathways, could also result in unexpected alterations in exposure to active species. In designing plans for regional therapy, such as intrathecal or intra-arterial drug administration, it is important to remember that agents requiring activation at a distant site will not be useful.
Table 44.3 Agents That Function as Prodrugs or Have Active Metabolite Species | |||||||||||||||||||||||||||
---|---|---|---|---|---|---|---|---|---|---|---|---|---|---|---|---|---|---|---|---|---|---|---|---|---|---|---|
|
As mentioned, most antimetabolites are activated at their target sties. For example, after entering cells, cytarabine is converted to the active nucleotide arabinosylcytosine triphosphate (Ara-CTP) in three phosphorylation steps catalyzed by deoxycytidine kinase, deoxycytidylate kinase, and nucleoside diphosphate kinase (104,105). Ara-CTP then acts as a fraudulent substrate for DNA replicative and repair enzymes. The pharmacokinetic parameters of the active intracellular metabolites are more predictive of response than plasma levels of the parent drug. For example, studies have shown little correlation between the pharmacokinetic parameters of cytarabine in plasma and leukemic cell concentrations of Ara-CTP. Ara-CTP retention in blasts correlates with response better than plasma drug concentrations (106,107,108). Furthermore, decreased deoxycytidine kinase activity, resulting in decreased accumulation of Ara-CTP, is a primary mechanism of Ara-C resistance (109,110,111,112).
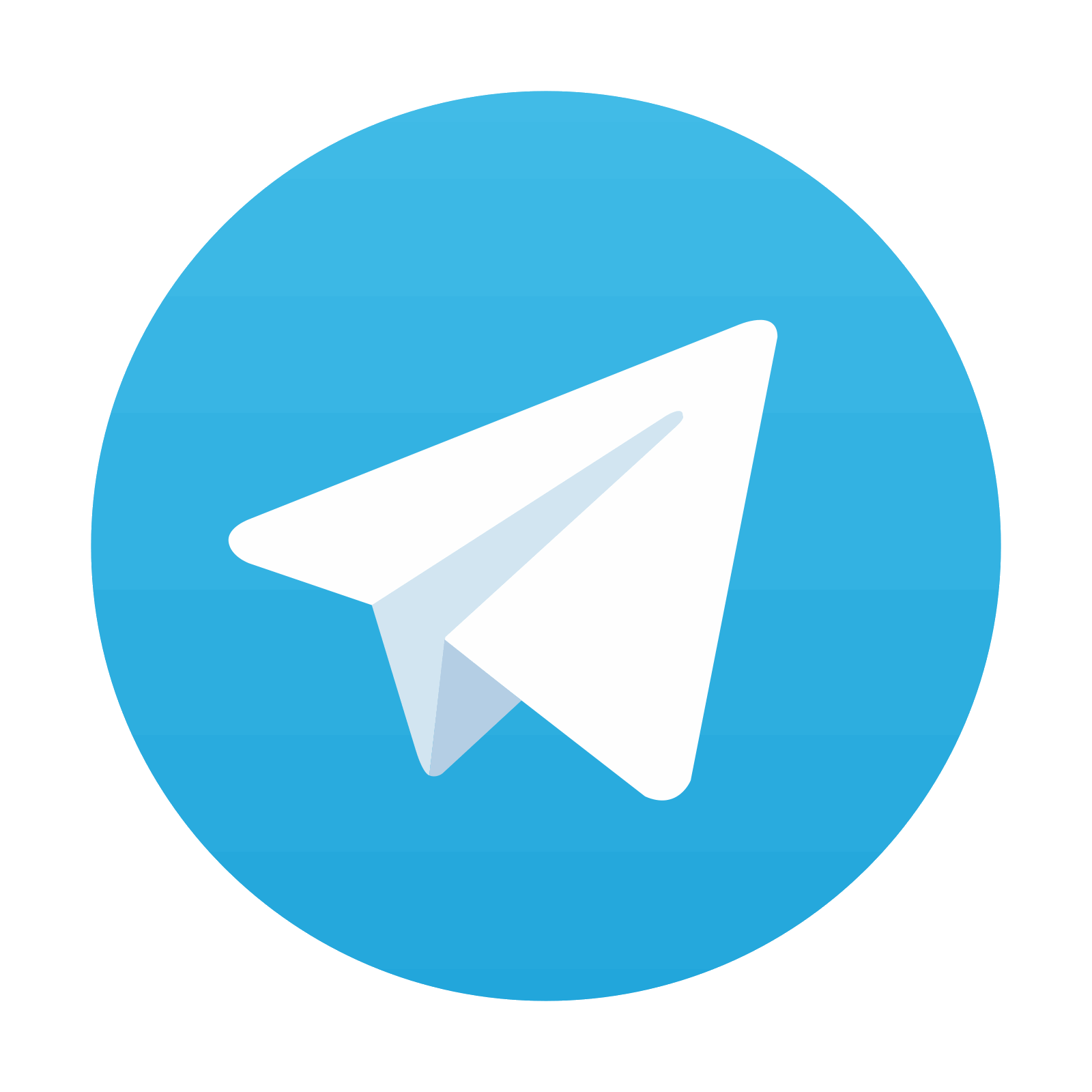
Stay updated, free articles. Join our Telegram channel
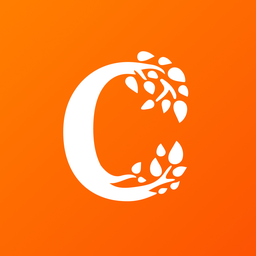
Full access? Get Clinical Tree
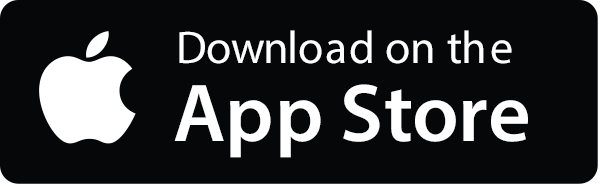
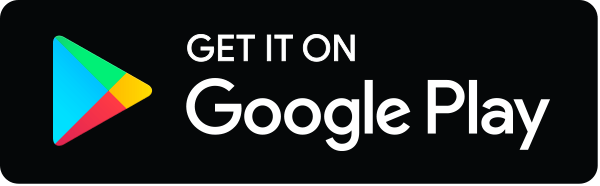