(5)
Department of Respiratory Care, Seattle Children’s Hospital, Foundation, Research Institute, Seattle, WA 98105, USA
Educational Goals
-
Describe a basic understanding of the oxygen transport chain.
-
Understand the various technologies for oxygenation monitoring during mechanical ventilation.
-
Describe the limitations associated with various monitoring technologies.
Karl Wilhelm Scheele first discovered oxygen in 1772.1 This immense milepost in the history of science and medicine set into motion events that have led us to the present in which the delivery and monitoring of oxygen is a mainstay of critical care. It is now possible to continuously measure many key aspects of the complex movement of oxygen molecules from the atmosphere to the lungs, to the blood, to the tissues, and finally, to the cellular structures that use oxygen and nutrients to create the energy molecules that fuel our biological engines. This chapter will focus on the most commonly used oxygenation monitoring techniques including their measurement principles, utility, and limitations. However, before we explore a brief review of what can be monitored, it is useful to consider what should be monitored. Sometimes, our technology develops at a pace that far exceeds our wisdom in how to use it. “New technologies and procedures have developed so rapidly—and there are such economic and social incentives to use them—that the evaluation of their safety, efficacy, and cost-effectiveness as well as the consideration of their social and ethical consequences have lagged far behind” (Mosteller and Institute of Medicine 1985). Another term for this is technology creep, which refers to the continuing addition of technology that is both qualitatively and quantitatively more complex. Moreover, this often happens without any rigorous testing of the effect of this technology on patient outcomes. Most new instrument/device testing mandated by regulatory agencies prior to approval is focused on ensuring patient safety and measurement accuracy and precision, as opposed to proving that the device in question will alter patient outcomes or improve processes of care.2
Some clinicians have adopted a “more is better” philosophy, wanting to monitor nearly everything possible (Hess 1998). Excessive numbers of monitors at the bedside can produce so much information for clinicians to process that the sheer volume can outpace their ability to analyze and integrate this information into timely decision making (East et al. 1991; Graham and Cvach 2010). There can be up to 40 different monitoring alarms assaulting the intensive care clinician (Chambrin 2001). It is reported that only 15 % of all monitor alarms in an intensive care environment are clinically relevant (Siebig et al. 2010).
Add this complexity to the fact that clinicians are often not adequately trained to understand the physiology and measurement principles related to the monitors they are using. In a survey of more than 4,300 responders, 70 % of neonatal care providers acknowledged that they do not have a complete education in oxygenation and have insufficient knowledge of basic concepts (Sola et al. 2008). In the same survey, 92 % did not know how SpO2 monitors work and the differences between various brands of SpO2 monitors. Knowledge (or the lack thereof) about pulse oximetry has been tested among pediatric clinicians, and the results have mostly been disappointing (Teoh et al. 2003; Popovich et al. 2004; Elliott et al. 2006).
To better understand the way in which oxygenation is monitored, the clinician must first understand the basic physiology of oxygen transport in the blood. In healthy humans, every 100 mL of arterial blood carries about 20 mL of oxygen at sea level. At rest, the body extracts about 5 mL of oxygen per 100 mL of arterial blood. This reserve allows for adequate tissue oxygenation during periods of high oxygen demand (i.e., exercise) or periods of low oxygen delivery (i.e., pulmonary and/or cardiac disease, or high altitude).
Entering the arterial blood in gaseous form, oxygen diffuses across the alveolar capillary membrane and dissolves into the plasma. But the oxygen carrying capacity of plasma alone is insufficient to sustain life. In 100 mL of plasma (with no hemoglobin), at a partial pressure of oxygen of 100 mmHg, there is only 0.3 mL of dissolved oxygen. Once dissolved in the plasma, oxygen quickly binds with hemoglobin molecules on the erythrocytes. The remarkable stereochemical properties of the hemoglobin molecule render it capable of binding to, carrying, and off-loading much more oxygen than can be dissolved in plasma alone. Once the erythrocytes have been transported through the arterial system to the capillary beds, the oxygen off-loads from the hemoglobin back into the plasma and thus diffuses across the capillary membranes into the adjacent tissues and finally through the cell membrane and into the mitochondria. Figure 12.1 visually describes some of the complex mechanisms involved in oxygen transport from the atmosphere to the tissues.
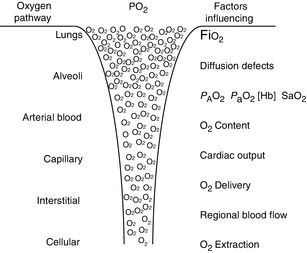
Fig. 12.1
Visual representation of the oxygen movement of oxygen from the atmosphere to the cellular level. CO cardiac output, SVR systemic vascular resistance (Used with permission from Sola et al. (2008))
The movement of oxygen molecules through our biological systems is governed by pressure gradients. The atmosphere has a higher partial pressure of oxygen than the plasma, which is higher than the interstitial partial pressure and so on. This is illustrated in Fig. 12.2. Oxygen transport to the cells depends on the partial pressure of oxygen in the alveoli, the amount of hemoglobin in the blood, the degree to which the hemoglobin is saturated, and the cardiac output. There can also be regional variations in blood flow that can affect oxygen transport. Finally, the diffusion of oxygen out of the plasma across the capillary membranes, into the interstitial space, into the cell, and finally into the mitochondria can be affected by interstitial fluid balances, capillary permeability, and anything else that increases the distance oxygen has to travel from the capillary wall to the adjacent cells.
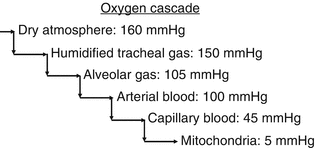
Fig. 12.2
The oxygen cascade is a conceptual rendering of the movement of oxygen from the atmosphere to the mitochondria down a descending pressure gradient. The partial pressure of oxygen in various parts of the oxygen transport chain is displayed. Various types of oxygenation monitoring systems monitor different parts of this cascade either directly or indirectly
Blood gas studies assess oxygenation by measuring the partial pressure of oxygen dissolved in the plasma alone (PO2). But this is only a small part of the oxygen transport chain. The utility of blood gas measurements are based on assumptions about the relationship between the partial pressure of dissolved oxygen in plasma and the amount of oxygen bound to hemoglobin. This relationship is described by oxyhemoglobin dissociation curve (Fig. 12.3). The curve is sigmoid shaped to facilitate that loading of oxygen onto hemoglobin in the lungs and off-loading oxygen from the hemoglobin in the somatic capillaries. Many factors affect the shape and position of the dissociation curve. These include temperature, pH, chronic hypoxemia, dyshemoglobinemias, and the availability of 2,3 diphosphoglycerate (2,3-DPG), a compound that affects the affinity of oxygen for hemoglobin (Hsia 1998). Decreased levels of 2,3-DPG are found in cases of erythrocytosis and nonspherocytic hemolytic anemia and 2,3-DPG phosphatase deficiencies. The effect of transfusion of stored RBCs on oxygen delivery is complex, and views about its effect are divergent. Historically, it was reported that 2,3-DPG levels were depleted in stored RBCs, but others have found no relationship between the length of time of storage of RBCs and biochemical markers of oxygenation in the critically ill (Tinmouth et al. 2006; Ibrahim et al. 2005a; Walsh et al. 2004). Increased levels of 2,3-DPG are found in conditions in which the body needs more oxygen, such as obstructive lung disease, cystic fibrosis, congenital heart disease, and hyperthyroidism. High altitudes and participating in exercise sessions can also elevate 2,3-DPG levels.
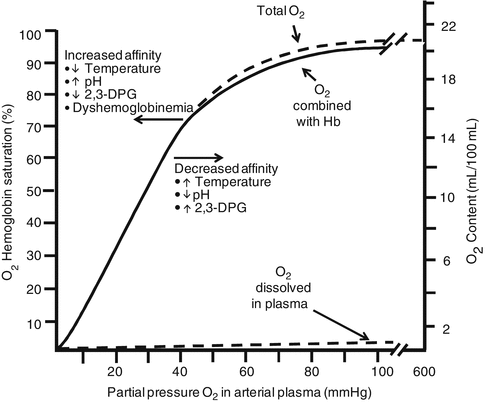
Fig. 12.3
Oxyhemoglobin dissociation curve. Note that the overwhelming majority of the total oxygen content is carried as oxygen bound to hemoglobin. The curve will shift left or right as affinity of oxygen for hemoglobin increases or decreases. Changes in temperature, pH, and levels of 2,3-diphosphoglycerate cause the affinity of oxygen for hemoglobin to change. Increased affinity is associated with decreased availability of oxygen to tissues (Brashers 2002). Recent work has called into question some of the assumptions about the relationship between pH and 2,3-DPG (Ibrahim et al. 2005b)
In general, those factors that shift the curve to the right are advantageous to the patient since this reflects a reduced affinity of oxygen for hemoglobin resulting in more oxygen released from hemoglobin to the tissues (Peter et al. 1991). The position of the curve is usually expressed by measurement of P50, which is defined as the PaO2 at which the hemoglobin is 50 % saturated with oxygen at a pH of 7.40, PaCO2 of 40 mmHg, and temperature of 37 °C.
It has been known for many years that there is large variation in affinity of oxygen for hemoglobin and thus the shape and position of the dissociation curve (Gøthgen et al. 1990; Wilkinson et al. 1980). No less than Ole Siggaard-Andersen, one of the principle developers of blood gas technology has said (Gøthgen et al. 1990), “…it is essential to know the actual position of the hemoglobin-oxygen dissociation curve, as well as the hemoglobin concentration in the individual patient, for correct interpretation of PO2……. in arterial blood.” Yet, historically, we have relied heavily on P aO2 alone for assessing oxygenation. Unfortunately, the actual measurement of p50 is not widely available and requires the tonometry of blood. There are calculators for P50, but these are based on assumptions about oxygen hemoglobin affinity which can vary widely (Gøthgen et al. 1990).
As clinical practice has evolved, the focus on P aO2 as the most widely used measure of oxygenation has been enhanced by the appreciation that SaO2 is a broader assessment of the oxygen transport chain. This value assesses a much larger portion of the mechanism of oxygen transport. It is also clear that a thorough assessment of oxygenation cannot be made without knowing the total serum hemoglobin. This can be seen when one considers the equations for oxygen content and oxygen delivery. These equations describe the volume of oxygen in a given amount of whole and the volume delivered (or available) to the tissues by the cardiovascular system:
Oxygen content (the volume of oxygen (in mL) carried in 100 mL of whole blood):
where

CaO2
oxygen content of arterial blood, expressed as mL/dL (normal values are 16–22 mL/dL)
Hb
total serum hemoglobin in g/dL
1.34
the volume of oxygen (in mL) that can be carried by a gram of hemoglobin that is fully saturated with oxygen
SaO2
the degree to which the hemoglobin is saturated in percent which should be expressed as a decimal for the mathematics of the formula
P aO2
partial pressure of oxygen in arterial blood in mmHg
0.0031
the oxygen solubility coefficient in plasma (the number represents the mL of oxygen that will be dissolved in plasma for each mmHg of partial pressure of oxygen dissolved in plasma)
Oxygen delivery:
where

DO2
oxygen delivery expressed as mL/min
CaO2
oxygen content of arterial blood expressed as mL/dL
CO
cardiac output expressed in mL/min
These formulae and other measures and derivatives of oxygenation, and their normal values are listed in Table 12.1. Careful review of these formulae reminds us that oxygen delivery is dependent on many factors including serum hemoglobin levels, cardiac output, oxygen saturation, and P aO2.
Table 12.1
Measures and derivatives of oxygenation
Measures |
Normal range | |
---|---|---|
Partial pressure of arterial oxygen (P aO2) |
80–100 mmHg | |
Partial pressure of arterial CO2 (P aCO2) |
35–45 mmHg | |
Arterial oxygen saturation (SaO2) |
95–100 % | |
Mixed venous saturation (SvO2) |
60–80 % | |
Arterial oxygen content (CaO2) |
(Hb × 1.34 × SaO2) + (0.0031 × P aO2) |
17–20 mL/dL |
Venous oxygen content (CvO2) |
(Hb × 1.34 × SvO2) + (0.0031 × PvO2) |
12–15 mL/dL |
A–V oxygen content difference (C[a − v]O2) |
CaO − CvO2 |
4–6 mL/dL |
Oxygen delivery (DO2) |
CaO2 × CO × 10 |
950–1,150 mL/min |
Oxygen delivery index (DO2I) |
CaO2 × CI × 10 |
500–600 mL/min/m2 |
Oxygen consumption (VO2) |
(C(a − v)O2) × CO × 10 |
200–250 mL/min |
Oxygen consumption index (VO2I) |
(C(a − v)O2) × CI × 10 |
120–160 mL/min/m2 |
Oxygen extraction ration (O2ER) |
[(CaO2 − CvO2)/CaO2] × 100 |
22–30 % |
Oxygen extraction index (O2EI) |
[SaO2 − SvO2]/SaO2 × 100 |
20–25 % |
12.1.1 Effects of Altitude
As altitude increases, barometric pressure decreases. Thus, even though the concentration of oxygen in the atmosphere is the same regardless of altitude, the partial pressure of oxygen decreases at increasing heights above sea level (Gallagher and Hackett 2004). As the P aO2 decreases, so does the SaO2. Figure 12.4 demonstrates the effect of altitude on the pressure of inspired oxygen (P IO2), P aO2, and SaO2 (Hackett et al. 2001).
The effect of altitude on “normal” pulse oximetry readings in infants and children has been studied (Beebe et al. 1994; Niermeyer et al. 1993, 1995; Thilo et al. 1991; Gamponia et al. 1998). Thilo reported that at an altitude of 1,610 m, healthy newborns had a mean SpO2 of 92–93 % and that the lower end of the reference range was as low as 86 % during quiet sleep at 1–3 months of age (Thilo et al. 1991). Niermeyer et al. studied serial SpO2 at an altitude of 3,100 m, measured from birth to 4 months in healthy infants. SpO2 ranged from 80.6 ± 5.3 to 91.1 ± 1.7 % during the 4 months period (Niermeyer et al. 1993).
The clinician is challenged with knowing how low SpO2 must go before supplemental oxygen is indicated at various altitudes. Subhi and colleagues did a systematic review of published normal SpO2 for children at various altitudes above sea level (Subhi et al. 2009). Tables 12.2 and 12.3 and Fig. 12.4 are adapted from their work. Figures 12.4 and 12.5 offers threshold values below which a child may be considered hypoxemic adjusted for altitude and thus requiring supplemental oxygen. The risk of the development of retinopathy of prematurity in low-birth-weight infants requires that different target values for SpO2 be used. See the section below on pulse oximetry.
Table 12.2
Normal (reference) ranges for blood gases in neonatal and pediatric populations
Age range |
Neonatal |
Pediatric | |||
---|---|---|---|---|---|
Source |
Arterial |
Venous |
Capillary (Cousineaua et al. 2005) |
Arterial |
Venous |
pH |
7.35–7.45 |
7.31–7.41 |
7.31–7.47 |
7.35–7.45 |
7.31–7.41 |
PCO2 |
35–45 mmHg |
41–51 mmHg |
29–49 mmHg |
35–45 mmHg |
41–51 mmHg |
PO2 |
50–90 mmHg |
30–40 mmHg |
33–61 mmHg |
80–100 mmHg |
30–40 mmHg |
Table 12.3
Normal pulse oximetry values for children 1–5 years of age at various altitudes
Age of study group |
Number of children studied |
Altitude above sea level (m) |
Reported SpO2 mean (SD) |
---|---|---|---|
2–60 months |
153 |
3,750 |
88.9 (2.9) |
2–23 months |
80 |
1,500 |
98.9 (1.0) |
0–2 months |
302 |
1,584 |
97.1 (2.7) |
1–60 months |
151 |
1,600 |
95.7 (2.7) |
1 month |
100 |
1,610 |
93.4 (2.0) |
7 days–36 months |
87 |
1,670 |
95.7 (1.6) |
0–24 months |
189 |
2,640 |
93.3 (2.1) |
0–18 months |
55 |
2,600 |
93.2 (3.0) |
0–60 months |
150 |
2,800 |
92.0 (2.0) |
2 days–22 months |
72 |
2,800 |
91.7 (2.1) |
0–60 months |
152 |
4,018 |
87.8 (3.8) |
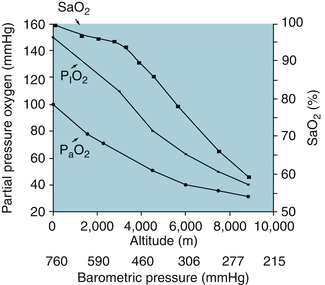
Fig. 12.4
Relationship between altitude, barometric pressure, pressure of inspired oxygen (P IO2), P aO2, and SaO2 (Adapted with permission from Hackett et al. (2001))
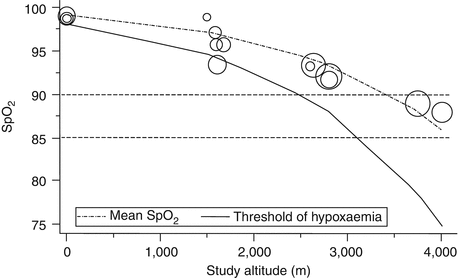
Fig. 12.5
Estimated threshold of hypoxemia at different altitudes. Study estimates are plotted as circles, with size proportional to the precision of the transformed study estimate (Used with permission from Subhi et al. (2009))
12.1.2 Blood Gas Measurements
12.1.2.1 Intermittent
Intermittent sampling and analyses of the pH, PCO2, and PO2 of arterial, venous, or capillary blood are some of the most frequently ordered laboratory tests. There have been tremendous advances in blood gas technology including miniaturization of components, improved quality control, simplified maintenance techniques, reduced sample size, decreased throughput time, and portability. But the measurement principles for blood gases remain the same. Blood gas machines actually measure only three variables, pH, PCO2, and PO2. All other variables reported on a blood gas, i.e., base excess, bicarbonate, and oxygen saturation,3 are calculated.
Blood gas machines use electrochemical sensors which are covered with selectively permeable membranes that allow the passage of either O2 or CO2. These gases then chemically react with reagents in their respective sensors to create voltages that are proportional to the amount of the respective gas that has entered the electrode, which correlates with the partial pressure of gas in the blood that has come in contact with the semipermeable membranes.
Advantages of intermittent blood gas sampling include well-known accuracy and precision, simplicity, convenience, relatively low cost, and a generally good understanding of the basics of blood gas measurement. Typically, PO2 electrodes are less accurate and precise than PCO2 electrodes. Intra-instrument imprecision4 is much improved in newer models of blood gas analyzers. For PO2 samples <150 mmHg, most instruments can produce measurements within ±2 % (Hansen et al. 1989; Scuderi et al. 1993). This imprecision worsens in PO2 > 150 mmHg.
Disadvantages include the need for an invasive blood sample, either through repeated percutaneous arterial or capillary sticks. Patients on ventilators for many days, can have much bruising and skin injury from repeated sticks, which can be very disturbing to parents, even though they typically heal without difficulty. Arterial lines obviate the need for repeated sticks, but in infants and children, utilization of arterial lines is not as prevalent as in adults. This is probably related to many factors including the pain of insertion, the limitations of the size of the patients, the risk of infection and mishap, and the known sequela of arterial lines.
From the early days when 10 mL of blood and 45 min was required to obtain and analyze a sample, we now have instrumentation that allows for blood gas measurements on far less than 1 mL of blood with results available within 90 s using handheld point of care analyzers. Whether or not having all blood gas results this rapidly ultimately affects patient outcomes remains largely unproven (Giuliano and Grant 2002).
Kendall and colleagues (1998) randomized 1,728 patients to be managed in the emergency room using either point of care or central laboratory testing. They found that point of care testing (which included blood gas, hematological, and chemical studies) reduced the time until lab results were available (for blood gases, a 20 min reduction). But they deemed that change in management in which timing was critical involved only 7 % of all samples. Moreover, there was no difference between groups in hospital admission rate, length of stay in the emergency department, hospital length of stay, and mortality.
In a very interesting study, Thomas and colleagues (2009) compared P aO2 and P aCO2 values from a point of care testing system to values obtained from a central laboratory with regard to running a ventilator management algorithm in 446 ventilated adult intensive care patients. In other words, would the recommendations of the ventilator management algorithm be different when using point of care values or central laboratory-derived blood gases? They concluded that the use of point of care blood gases versus central blood gas laboratory values produced equivalent ventilator management algorithm recommendations. Their study included no cost analyses.
Despite increased proliferation of handheld, point of care blood gas analyzers, there remains tremendous variation in the use of these devices. Some intensive care units rely exclusively on point of care testing for blood gas analysis. Others use little or no point of care testing, relying instead on near patient, bench blood gas analyzers, or analyses done in a central laboratory.
A search has been underway for more than 10 years to prove that point of care testing is cost effective. Results have been divergent depending on what type of point of care testing is being studied (O’Connell et al. 2008; Macnab et al. 2003; Englander et al. 2006). Clearly, there are some cases where immediate testing results at the bedside seem likely to make a meaningful difference in outcomes, such as in surgery, during ECMO, or during transport. But my observations have led me to conclude that in a typical intensive care setting, the vast majority of point of care blood gas testing is not emergent and that results within 15–30 min would be completely acceptable. Additionally, any potential savings of implementing point of care blood gases systems would be dependent on elimination of the instrumentation and labor costs of other blood gas analysis systems. Most applications of point of care blood gas testing I have seen simply layered the point of care capabilities on top of existing laboratory blood gas systems, resulting in cost increases with no proven effect on patient outcomes. Most of the published financial analyses of the impact of point of care testing are not sophisticated and often confuse the cost of the blood gases with the charges for blood gases. The interested learner is directed to a thorough review of the general topic of point of care testing by Kost (1998).
Many blood gas instruments do not actually measure SaO2, but instead calculate (or estimate) the SaO2 based on assumptions about the shape of the oxygen dissociation curve. Wilkinson et al. showed that in some newborns, when P aO2 was less than 50 mmHg, calculated SaO2 could be >90 %, whereas in others <75 % (Wilkinson et al. 1980). My colleagues and I showed that in pediatric ICU patients, calculated oxygen saturation levels were unreliable, particularly when true saturation levels were <90 % (Salyer et al. 1989). Clinicians are advised not to rely on calculated oxygen saturation values derived from blood gas measurements.
The most common method of direct laboratory measurement of SaO2 requires the use of a CO-oximeter. This name can be a little misleading since it was derived from early instruments designed to measure carboxyhemoglobin (HbCO). Laboratory CO-oximeters use multiple wavelength spectrophotometry of hemolyzed blood samples to measure SaO2. This measurement principle is basically the same as is used in pulse oximetry, which we will discuss in detail later. Typically, point of care blood gas tests do not actually measure SaO2 so care must be taken in interpreting low saturation values from point of care devices. Some of the latest bench blood gas analyzers incorporate spectrophotometry into their measurement capabilities and thus can report actual measured SaO2. The interested clinician should check and ensure that the SaO2’s reported with blood gas results are actually measured using CO-oximetry versus calculated.
12.1.2.2 Continuous Blood Gas Monitoring
Continuous intravascular monitoring of blood gases became widely available in the 1990s and has been steadily improving since then (Ganter and Zollinger 2003). This technology uses various techniques to miniaturize blood gas measurement sensors sufficiently to allow them to be imbedded in a catheter that can be placed in the radial, brachial, and femoral artery for continuous monitoring. Measurement principles may be electrochemical (as in bench blood gas analyzers) or photochemical/optical (also called optodes). Optodes use sample chambers that contain dyes which are illuminated with light of a certain wavelengths. The illuminating light will be transmitted, reflected, absorbed, and reemitted proportionally to the concentration of oxygen, carbon dioxide, and hydrogen ions in the sample.
The radial artery is the most common site for catheter insertion in adults and the femoral artery in children <5 years old. The disadvantages of the radial approach include increased susceptibility to motion artifact, vasospasm, and changes in peripheral blood flow. Nevertheless, this approach is chosen routinely in adults and older children, because of easy access, the double blood vessel supply of the hand, and low complication rates. For newborns, the umbilical artery is used.
Proponents of this technology argued that substantial changes in arterial blood gases can be missed by intermittent blood gas analyses (Zaugg et al. 1998). Moreover, it is suggested that continuous blood gas monitoring could (1) allow for more rapid identification of changing trends in blood gases, (2) decrease therapeutic decision time, (3) reduce blood loss from repeated sampling, and (4) make it possible to use the continuous blood gas results for closed loop control of ventilator settings using feedback-control algorithms (Mahutte 1998). As is the case with so much new technology, little systematic investigation has been done to validate these assumptions.
These systems are very costly. The instruments themselves cost as much as a bench blood gas analyzer and yet can only be used on one patient at a time. The catheters are also very costly, and some patients end up needing more than one because of catheter failure. They are also very invasive and prone to technical problems such as kinked or clotted catheters and problems with poor regional blood flow. In my experience in the USA visiting many pediatric intensive care units, I have seen very few in use.
12.1.3 Pulse Oximetry
One of the more remarkable advances in oxygenation monitoring technology is the development of the pulse oximeter (Salyer 2003). Pulse oximeters use principles of spectrophotometry to estimate arterial oxygen saturation noninvasively using sensors that can be clipped to an ear or finger or, for smaller patients, can be wrapped around digits, palms, and feet. Pulse oximetry estimates arterial oxygen saturation by measuring the absorption of light of two wavelengths, approximately 660 nm (red) and 940 nm (infrared), in human tissue beds. As light passes through human tissue, it is absorbed in various degrees by the skin, tissues, bone, fluids, and blood. The amount of blood in the tissue beds changes with each beat of the heart. This change in volume causes a change in spectral absorption, which the oximeter can detect and thus estimate heart rate. As the relative amounts of oxygenated and deoxygenated hemoglobin changes in the tissue bed, the spectral absorption also changes, and thus the oximeter can estimate SaO2.
The accuracy of pulse oximetry has been studied extensively in various populations (Choe et al. 1989; Morris et al. 1989; Faconi 1988; Hay et al. 1989; Nickerson et al. 1988; Severinghaus et al. 1989; Hannhart et al. 1991; Taylor and Whitman 1988; Wouters et al. 2002a, b; Deckardt and Steward 1984; Jennis and Peabody 1987; Walsh et al. 1987; Southall et al. 1987; Solimano et al. 1986; Ramanathan et al. 1987; Praud et al. 1989; Boxer et al. 1987; Carter et al. 2001) and is generally about ±1–2 % of readings >85 % (Severinghaus 1993; Emergency Care Research Institute 2003; Van de Louw et al. 2001). During periods of profound desaturation (typically below 70–80 %), bias and precision of oximeters deteriorates significantly, being highly variable depending on brand (Faconi 1988; Severinghaus et al. 1989). This limitation has never been particularly problematic for most clinicians since an SpO2 of 40 % versus 60 % will illicit the same aggressive interventions to improve oxygenation.
As we have learned more and more about the behavior of oximeters during continuous monitoring, it has become apparent that there are serious performance problems with some types of pulse oximeters that are not related to accuracy, but instead to precision and reliability, e.g., the ability of the devices to produce credible readings over time. More about this are discussed below.
Continuous monitoring with a pulse oximeter was originally mostly confined to the perioperative period. But it has now grown in many places to include all intensive care patients, any child on oxygen, anyone undergoing procedural sedation, anyone presenting with respiratory symptoms in the emergency department, or anyone on patient-controlled anesthesia, to name a few. Indeed, SpO2 readings have become a de facto fifth vital sign. In terms of processes, pulse oximetry has been shown to sometimes reduce the number of arterial blood gas samples obtained in various populations (King and Simon 1987; Kellerman et al. 1991; Inman et al. 1993; Bourdelles et al. 1998; Roizen et al. 1993; Niehoff et al. 1988).
But as the use of pulse oximetry spread, little evidence was developed demonstrating the effect of continuous monitoring with a pulse oximeter on outcomes of care. It has been said that “absence of evidence is not evidence of absence.”5 Many technologies have not been thoroughly tested, and thus their effect on outcomes has been suspect. But it is risky to then build arguments based on the assumption that this absence of evidence is a result of having looked for it and not found it. But in the case of oximetry, there has been a decade long search for proof that continuous monitoring changes the outcomes of care. For most of this time, no such effect has been found (Ochroch et al. 2006; Pedersen et al. 2009).
Moeller et al. enrolled more than 20,800 surgical patients and randomized them to receive continuous pulse oximetry during the perioperative period. The two groups did not differ significantly in cardiovascular, respiratory, or neurologic complications; length of hospital stay; or in-hospital deaths (Moller et al. 1993a).
It is possible that not using the pulse oximeter and, thereby, not identifying and treating episodic hypoxemia might have more subtle effects on the higher brain functions of memory and cognition. In a follow-up study, Moeller and colleagues studied this question by randomizing 736 surgical patients to continuous pulse oximetry during the perioperative period (Moller et al. 1993b). Cognitive function was evaluated before surgery, at 7 days and 6 weeks following surgery. The authors concluded that “. . . subjective and objective measures did not indicate less postoperative cognitive impairment after perioperative monitoring with pulse oximetry.”
Bowton et al. studied (1991) the effect of continuous pulse oximetry on a population of general medical–surgical patients. Reviews of the oximeter records were compared with the nurses’ charting, doctors’ progress notes, and doctors’ orders. Desaturations that were identified by the pulse oximeter were noted in the nursing notes only 33 % of the time and in the doctors’ progress notes 7 % of the time. Changes in the orders for respiratory therapy were noted in only 20 % of patients who desaturated to <85 %.
A Cochrane systematic review of the effect of pulse oximetry in the perioperative period by Pedersen et al. (2009) concluded, “…studies confirmed that pulse oximetry can detect hypoxaemia and related events. However, we have found no evidence that pulse oximetry affects the outcome of anaesthesia for patients. The conflicting subjective and objective results of the studies, despite an intense methodical collection of data from a relatively large general surgery population, indicate that the value of perioperative monitoring with pulse oximetry is questionable in relation to improved reliable outcomes, effectiveness, and efficiency. Routine continuous pulse oximetry monitoring did not reduce either transfer to ICU or mortality, and it is unclear if there is any real benefit from the application of this technology in patients who are recovering from cardiothoracic surgery in a general care area.”
However, both my observations of how oximeters are actually used and a careful reading of the literature lead me to conclude two important things about the impact of continuous pulse oximetry on patient outcomes. First, not all oximeters perform the same, and second, an oximeter will not substantially alter patient care unless it is used by people who are thoroughly trained and in conjunction with a systematic methodology for how to set oximeter alarms and how to react to oximeter alarms. We know that clinicians are generally not very well trained in exactly what a pulse oximeter measures, and they know very little about performance differences between brands (Sola et al. 2008; Teoh et al. 2003; Popovich et al. 2004). And collectively, we are beginning to understand that superimposing additional technology on top of unreliable clinical processes will not necessarily improve the process at all, but may instead simply create a more technologically advanced unreliable process (Stapleton et al. 2009; Thompson et al. 2003).
Anyone who has long worked in the quality assurance field of health care will appreciate that policy and practice may often not be well acquainted. In the case of pulse oximeter alarm limits, this is particularly true. It has been shown that compliance with pulse oximetry alarm limit policies is as low as 9 % of patients (Clucas et al. 2007). This has certainly been my clinical experience. This is probably largely due to the propensity of some brands of pulse oximeters to generate lots of false desaturations. The clinicians are simply setting the alarm limits at levels that will keep the oximeters from driving them to distraction. Even so, there is still a great deal of alarm desensitization because of the poor performance of some brands of pulse oximeters. This noncompliance is also probably a contributing factor in why it was originally difficult to show an influence of pulse oximetry on patient outcomes. But as technology and clinical processes have improved and standardized, the impact of pulse oximetry has become more detectable.
As an example, Durbin and Rostow demonstrated that using motion-resistant6 pulse oximetry technology in conjunction with a standardized process of postoperative weaning resulted in a reduction in oximeter failure rates, arterial blood gas utilization, and oxygen weaning time in adult cardiovascular surgery patients when compared to a conventional pulse oximeter (Durbin and Rostow 2002).
Chow et al. also demonstrated improved outcomes associated with the implementation of an oxygen management protocol while switching to motion-resistant pulse oximetry (Chow et al. 2003). In this study of low-birth-weight infants, retinopathy of prematurity rates went from 12.5 to 2.5 % after introduction of new pulse oximeter technology and a rigorous oxygen management protocol.
Taenzer et al. reported that unplanned transfers to the intensive care unit decreased by 48 % after introduction of motion-resistant pulse oximetry and a centralized monitoring system7 to an orthopedic unit (Taenzer et al. 2010). This system sends all pulse oximetry data via wireless technology to a central computer server that notifies nurses by pager when their patients desaturated.
12.1.3.1 Limitations of Pulse Oximetry
Not all pulse oximeters are created equal. Although the accuracy of pulse oximeters has been demonstrated repeatedly, there are serious issues with the ongoing precision and reliability of the readings produced. Most studies of oximetry performance have focused on comparing single SpO2 readings to the SaO2 of simultaneously drawn arterial blood samples that are measured in a CO-oximeter. When this is done, accuracy is typically ±2 % (actual reading). The more important issue for monitoring critically ill patients is the reliability of continuous readings over time. False alarms can be a major problem in critical care units (Lawless 1994; Bohnhorst and Poets 1998). It turns out that in neonatal, pediatric, and adult populations, some pulse oximetry technology is prone to increased false desaturation readings and data dropout, which is defined as the amount of time the pulse oximeters display no reading at all.
In infants and children, the most common limitation is the effect of motion and low perfusion (Petterson et al. 2007). As children move the extremity being monitored, wiggling their fingers and toes, or experience periods of low perfusion, the absorption being measured by the oximeter is constantly changing in an environment where the signal-to-noise ratio of the changes in absorption is very low. These changes can be misinterpreted by oximeters as false desaturations or even cause some oximeters to display no readings at all (data dropout). Some pulse oximeters use sophisticated signal processing algorithms to filter and analyze these signals and determine through statistical modeling if the readings are reliable enough to be displayed. This is typically called motion resistance, and it is claimed by a number of manufacturers (Bohnhorst and Poets 1998). The computer in the oximeter must decide if the data at hand should be displayed, and the complex confidence arbitration algorithms for this are proprietary and thus the exact details of how this is done are often not available. How well these various algorithms work is a point of great debate among manufacturers.
The clinicians must also be aware that pulse oximetry software is frequently changing, and although your hospital may have only one brand of oximetry, you may have many different software versions that perform differently. Another confounder when thinking about pulse oximeter performance is the misleading use of one manufacturer’s sensors by another manufacturer. As an example, if you buy Philips multiparameter monitoring systems, you can get many different software and hardware configurations. You can get their monitors equipped with Nellcor, Masimo, or Philips oximetry signal processing software. You can also use Nellcor probes with Philips signal processing. Thus, clinicians may look at the Nellcor sensor and assume they have Nellcor signal processing in their multiparameter, which they may not. Sometimes, the front-line manufacturer representatives are not forthcoming with this information.
Ahlborn et al. (2000) showed that the use of the motion-resistant pulse oximetry of Masimo Signal Extraction Technology (SET)8 in a population of low-birth-weight infants resulted in a 75 and 47 % reduction in false alarm rates compared to two other brands of oximeters.
Sahni and colleagues (2003) studied a population of 15 healthy infants during various activity states. They showed that the use of Masimo SET resulted significantly less artifact. Figures 12.6 and 12.7 show SpO2 and heart rate readings from the two brands of oximeters in these healthy infants during various activity states.
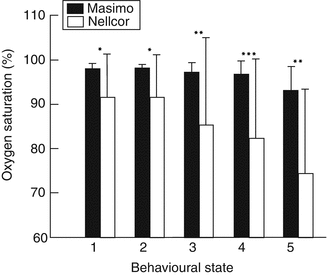
Fig. 12.6
Oxygen saturation measurements (means (SD)) with Masimo and Nellcor pulse oximeters in 15 healthy infants during different behavioral activity states: 1 quiet sleep, 2 indeterminate sleep, 3 active sleep, 4 awake, 5 crying. *p, 0.02; **p, 0.005; ***p, 0.0005 (Used with permission from Sahni et al. (2003))
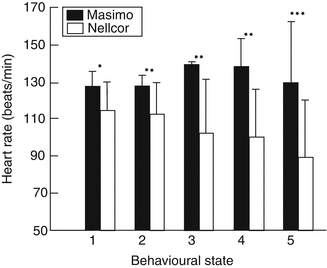
Fig. 12.7
Heart rate measurement (means (SD)) with Masimo and Nellcor pulse oximeters in 15 healthy infants during different behavioral activity states: 1 quiet sleep, 2 indeterminate sleep, 3 active sleep, 4 awake, 5 crying.*p, 0.005; **p, 0.0005; ***p, 0.00005 (Used with permission from Sahni et al. (2003))
In another study which compared neonatal transcutaneous PO2 (TCPO2) measurements to SpO2, Bohnhorst and colleagues showed that episodes of hyperoxia detected by TCPO2 were missed by the Masimo SET technology in only 0.5 % of episodes compared to 5.4 % by another brand (Bohnhorst et al. 2000).
In another study of the utility of various brands of pulse oximeters in neonates, Hay and colleagues (2002) showed that there were 86 % fewer false hypoxemias with the Masimo SET than other brands. Table 12.4 shows the results of this study for four different brands of oximeters. Data dropouts have also been shown to be much lower with the Masimo SET technology compared to some brands of oximeters (Workie et al. 2005).
Table 12.4
Incidence of performance problems of four new-generation pulse oximeters in a neonatal population
Masimo SET |
Nellcor N-395 |
Novametrix MARS |
Philips Viridia | |
---|---|---|---|---|
False hypoxemia |
1 |
42 |
35 |
10 |
Data drop outs |
1 |
10 |
95 |
21 |
Torres et al. compared Masimo SET technology to another brand in postoperative pediatric cardiopulmonary bypass patients and reported that the SpO2 failure rates were 10 % in the Masimo and 41 % in the other brand (Torres et al. 2004).
Another important limitation of pulse oximeters is presence of elevated levels of dyshemoglobins such as carboxyhemoglobin, methemoglobin, and sulfhemoglobin. These variants of hemoglobin arise out of various clinical conditions such as carbon monoxide inhalation, nitric oxide therapy, and the ingestion of certain poisons. These dyshemoglobins cannot bind adequately with oxygen and when elevated can cause a patient to have a very low true oxyhemoglobin desaturation in the presence of very high P aO2’s. Thus, relying on P aO2 alone, the clinician may have a false sense of security regarding the patient’s level of saturation. Table 12.5 demonstrates this phenomenon in a 12-month-old child. Nearly all currently available pulse oximeters cannot distinguish oxyhemoglobin from these dyshemoglobins and thus will read falsely high in the presence of elevated levels. To measure these, an oximeter would have used more than two wavelengths of light (as does a CO-oximeter). Masimo has marketed a pulse oximeter that purports to measure total serum hemoglobin, carboxyhemoglobin, and methemoglobin noninvasively. Little controlled scientific data has yet been subjected to peer review on this product. Early reports are promising (Annabi and Barker 2009; Barker et al. 2006; Rabe et al. 2010) but the technology needs to subjected to more testing under more challenging conditions in various populations.
Table 12.5
Pulse oximetry readings, P aO2, actual arterial saturation, and methemoglobin (MetHb) levels in a 12-month-old infant suffering from phenazopyridine hydrochloride ingestion
Time |
P aO2 (mmHg) |
SpO2 (%) |
SaO2 (%) |
MetHb (g/dL) |
---|---|---|---|---|
Admission |
155 |
63 |
44 |
55 |
4 h |
102 |
81 |
66 |
30 |
16 h |
110 |
89 |
82 |
15 |
24 h |
100 |
95 |
93 |
6 |
12.1.4 Summary
The movement of oxygen through the human body is a complex phenomenon. It is important for the clinicians to understand which part of the oxygen transport chain is being measured by various oxygenation monitoring technologies. Heavy reliance on P aO2 measurements alone may give a limited view of oxygenation monitoring. Other factors to consider are cardiac output, serum hemoglobin levels, and the oxygen saturation of hemoglobin. Normal oxygen saturation diminishes with increasing altitude.
Point of care blood gas testing has gained widespread acceptance without any compelling evidence that it has substantially altered patient outcomes.
Pulse oximetry has greatly simplified oxygenation monitoring and can be a powerful and convenient tool for oxygenation monitoring. However, there continue to be knowledge deficits of the clinicians regarding what oximeters actually measure and how the performance of oximeters vary between and within brands. While pulse oximetry accuracy has been well established, there are significant problems with the reliability of readings over time with some brands. Motion-resistant pulse oximetry (Masimo SET) has been shown to significantly reduce false desaturations and data dropout when compared to other pulse oximeters.
Essentials to Remember
-
The movement of oxygen through the human body is complex.
-
The most common measures of oxygenation, P aO2, and SaO2 only measure certain aspects of the oxygen transport system. A more thorough understanding of the oxygen transport system and oxygenation monitoring technology will help clinicians make decisions based on the data from continuous or intermittent oxygenation monitoring.
-
Point of care invasive oxygenation studies like blood gases have not been shown to substantially alter the outcomes of care when compared with centralized laboratory testing.
-
The most widely used measure of oxygenation is pulse oximetry.
-
There are important performance differences between brands of oximeters.
-
Motion-resistant oximeters are superior and reduce false desaturations, false alarms, and data dropout. When used with carefully controlled clinical protocols, they can also alter care processes and improve patient outcomes.
12.2 Ventilation: Adequacy of Breathing Assessment
Gerd Schmalisch6 and Ira M. Cheifetz7
(6)
Clinic of Neonatology, Charité – Universitätsmedizin Berlin, Charitéplatz 1, Berlin, D-10117, Germany
(7)
Division Pediatric Critical Care Medicine, Pediatric Intensive Care Unit, Pediatric Respiratory Care and ECMO, Duke Children’s Hospital, 3046, Durham, NC 27710, USA
Educational Aims
-
Understand the differences between time-based and volume-based (volumetric) capnography.
-
Review technologic considerations of capnography.
-
Understand the interpretation of the various phases of the time-based capnogram.
-
Discuss the unique aspects of carbon dioxide monitoring in the premature infant population.
-
Understand the interpretation of the phases of the single-breath carbon dioxide waveform associated with volumetric capnography.
-
Review the effects of both ventilation and perfusion on carbon dioxide elimination.
-
Discuss the various clinical applications of time-based and volume-based capnography.
-
Review the prognostic value of the dead space to tidal volume ratio (V d/V t).
12.2.1 Capnography
Noninvasive carbon dioxide monitoring provides valuable clinical information for the neonatal, pediatric, and adult patient populations. Capnography is an essential monitoring system for critically ill patients and is increasingly being described as the fifth vital sign.
Capnometry is the digital display of data, while capnography is the graphical display of data which can be presented time based or volume based (i.e., volumetric; see Sect. 12.2.3). Capnography refers to the visual depiction via waveforms of exhaled CO2 during the entire respiratory cycle and is a better indicator for dynamic changes in a patient’s gas exchange than capnometry. When capnography is used, proper clinical interpretation of the data and waveforms is essential to provide optimal management of the ventilator as well as safe patient care.
Time-based capnography is commonly known as end-tidal carbon dioxide (etCO2) monitoring. A time-based capnogram provides qualitative information on the waveform patterns associated with invasive ventilation and a quantitative estimation of the partial pressure of expired CO2. Volumetric capnography utilizes a CO2 sensor and a pneumotachometer in combination. This approach permits the calculation of the net volume of CO2 expired and is expressed as a volume exhaled per unit time (most commonly mL/min) rather than a partial pressure or gas fraction. Additionally, volumetric capnography allows for the calculation of the airway dead spaces, including the dead space to tidal volume ratio (V d/V t).
12.2.2 Time-Based Capnography/End-Tidal Carbon Dioxide Measurements
12.2.2.1 Methods, Waveforms, and Nomenclature
End-tidal carbon dioxide measurement is one of the primary variables obtained from time-based capnography. For these measurements, the required interface with the ventilator circuit is called a capnograph. Carbon dioxide elimination can be measured by either mainstream or sidestream technology.
Several studies, in particular in infants, have demonstrated that mainstream measurements are superior to sidestream measurements from the technical perspective (Pascucci et al. 1989). Mainstream sensors have a faster response time, such that reliable single-breath CO2 measurements, even at high respiratory rates, are possible. However, the additional apparatus dead space (V Dapp) of the CO2 analyzer chamber leads to rebreathing of the exhaled CO2, which should be considered; however, V Dapp is generally only clinically significant in very small infants. Mainstream sensors have become the more typical approach for capnography in mechanically ventilated larger infants, children, and adults.
Sidestream measurements utilize a sample port at the endotracheal tube (ETT) and are void of dead space; however, the suction flow rate required to sample exhaled gas may adversely affect measurement accuracy and response time. In neonates with a minute ventilation of about 200 mL/min/kg body weight, the suction flow must be sufficiently low to prevent dilution by surrounding air that will occur when the expiratory gas flow rate falls below the suction flow rate. A microstream capnograph using a suction flow of 30 mL/min and a miniaturized sample chamber has been developed, thus, improving the measurement accuracy for intubated infants (Hagerty et al. 2002; Kugelman et al. 2008).
Several techniques to measure the CO2 in expired air have been developed (Gravenstein et al. 2004). Currently, infrared (IR) spectrography and mass spectrometry (mainly for multiple gas analyses) are most frequently used. However, only IR spectrography utilizes miniaturized, low-cost main- and sidestream sensors optimized for measurements in the infant population. An alternative fast method could be the molar mass measurement of the breathing gas by ultrasound spirometry. Of note, ultrasonic flow meters have a very short response time without a time delay between the measured air flow and molar mass of the breathing gas. Theoretically, such devices are well suited for volumetric capnography in small lungs. However, molar mass techniques utilize a surrogate signal which is influenced by all components of the expired gas, and, thus, it is difficult to separate the CO2 signal (Thamrin et al. 2007).
Capnographs measure either the partial pressure (PCO2) (IR analyzer) or the CO2 fraction (FCO2) of the breathing gas (mass spectrometers). The relationship is given by Dalton’s law:
where P B is the barometric pressure and 47 mmHg is the water vapor pressure at 37 °C. However, with a dry gas (e.g., calibration gas from a gas cylinder), PCO2 is calculated by:

(12.1)
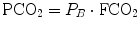
(12.2)
The time-based waveform of the measured PCO2 (or FCO2) is called a capnogram and can be divided into four phases as shown in Fig. 12.8. Phase I starts with inspiration and represents, after a short CO2 rebreathing, mainly the CO2-free inspired gas. Phase II starts with exhalation and consists of a rapid S-shaped upswing on the tracing due to mixing of dead space gas with alveolar gas. Phase III describes the so-called alveolar plateau representing CO2-rich gas from the alveoli. In older infants and adults, phase III almost always has a slightly positive slope, indicating a rising PCO2 due to the delayed emptying of alveoli with a low ventilation/perfusion ratio (high PCO2). In ventilated newborns, this slope is rarely seen due to their typically fast exhalation times. An exaggerated positive slope is seen in patients with pulmonary inhomogeneity, high airway resistance, and/or mechanical obstruction of the airway as alveoli empty carbon dioxide at a variable rate in these conditions. This is discussed in more detail below. Phase IV is actually a component of phase I and describes the decrease of the exhaled CO2 to zero at the beginning of inspiration.
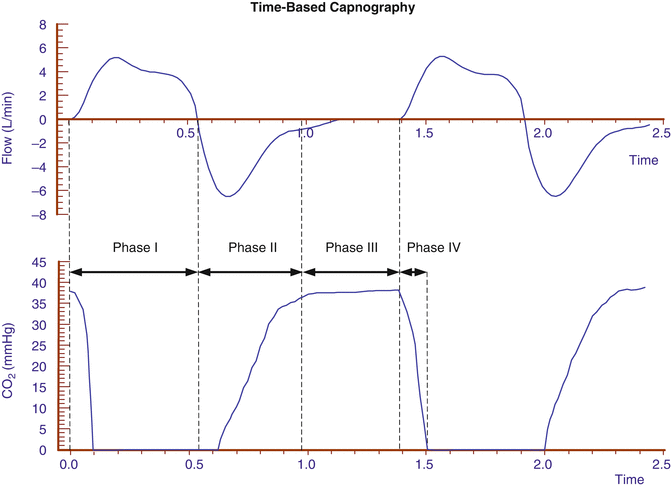
Fig. 12.8
A typical time-based capnogram of a ventilated neonate divided in 4 phases
12.2.2.2 P etCO2 Monitoring
Since capnography is noninvasive, its utilization to monitor gas exchange in ventilated infants, children, and adults is very attractive, and the most typical parameter monitored is end-tidal CO2 (P etCO2). The various factors that increase or decrease P etCO2 are summarized in Table 12.6 (Shapiro and Kacmarek 1998).
Table 12.6
Clinical factors that affect P etCO2 (Shapiro and Kacmarek 1998)
Increases PetCO2 |
Decreased PetCO2 | |
---|---|---|
CO2-production and delivery to the lung |
Increased by
• fever
• sepsis
• bicarbonate administration
• seizures | |
Decreased by
• hypothermia
• pulmonary hypoperfusion
• cardiac arrest
• pulmonary embolism
• hemorrhage
• hypotension | ||
Alveolar ventilation |
Decreased by
• increased deadspace
• hypoventilation
• ventilatory inhomogeneity | |
Increased by
• hyperventilation | ||
Equipment malformations |
• CO2-rebreathing due to apparatus deadspace | |
• ventilator disconnection
• esophageal intubation
• complete airway/ endotracheal tube obstruction
• poor gas sampling
• too low response time
• endotracheal tube leakage |
Under ideal conditions, P etCO2 accurately represents alveolar PCO2. However, this relationship is dependent on the delivery of carbon dioxide to the lung via the circulatory system (i.e., perfusion) and its removal from the lung (i.e., ventilation). Thus, the relationship between alveolar PCO2 and P etCO2 is a reflection of the ventilation/perfusion ratio (
) as assessed by the dead space to tidal volume ratio (V D/V T) (McSwain et al. 2010).

An important prerequisite for a P etCO2 measurement is the presence of a stable alveolar plateau at end expiration. Only if the capnogram shows such a plateau can we assume that the P etCO2 accurately reflects the alveolar PCO2. However, in ventilated infants and some ventilated children with increased airways resistance, this is not guaranteed as the adjusted expiratory time of the ventilator can be longer than the actual exhalation time of the patient (i.e., premature termination of exhalation). In such a situation, there is no patient flow at end expiration (Fig. 12.9), and, thus, P etCO2 cannot be determined (Proquitte et al. 2004). Confounding variables do exist in the neonatal and pediatric populations. The presence of large endotracheal tube air leaks makes the measurement of “end-tidal” values less reliable (Schmalisch et al. 2012). Additionally, significant patient–ventilator dyssynchrony (Fig. 12.10) can make the accurate determination of P etCO2 difficult.
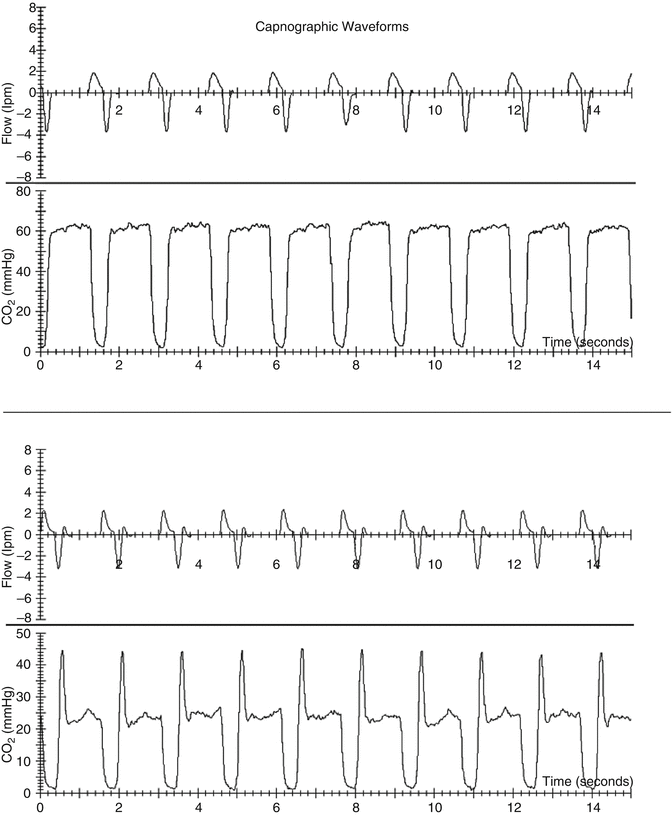
Fig. 12.9
Capnogram of a ventilated surfactant-depleted tracheotomized piglet with a stable alveolar plateau even during the expiratory pause (top) and a capnogram where P etCO2 was falsified by CO2 washout of the sample cell during the expiratory pause (bottom). Data from Proquitté et al. (2004)
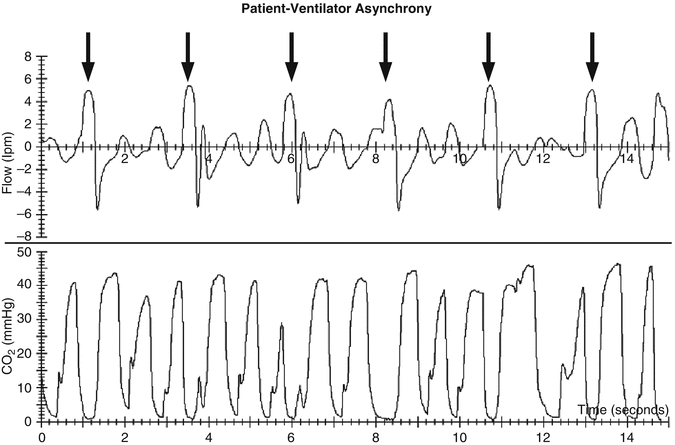
Fig. 12.10
Capnogram of a ventilated newborn with a mismatch of mechanical breath (black arrows) and spontaneous breathing
Therefore, P etCO2 measurements in ventilated patients are only useful if the shape of the capnogram is considered. Automated measurements of P etCO2 without monitoring the waveform should be used with caution as the resultant data can be misleading. Similarly, we should interpret P etCO2 values published in older literature with caution as the technical options for adequate monitoring of the capnogram were often very limited.
12.2.2.3 A Practical Approach to Interpreting Capnograms
What information can the capnogram offer the bedside clinician? At first, the presence of a physiologic meaningful P etCO2 should be assessed. If such a P etCO2 is not present, failure to ventilate the patient’s lungs must be assumed. Table 12.7 summarizes typical causes of absent CO2 elimination (Schmalisch 2004). Second, the shape of the capnogram must be compared with a typical pattern as shown in Fig. 12.8 by inspecting the inspiratory CO2 baseline, steepness of phase II, alveolar plateau of phase III, and decline of the capnogram at the beginning of the next inspiration. Third, depending on the capnograph and the monitoring software utilized, the clinician should assess the characteristic parameters derived from the capnogram, for example, P etCO2, CO2 elimination (VCO2), P a − etCO2, and dead space (anatomic and alveolar).
Table 12.7
Differential diagnostic causes of absent end-expiratory CO2
Patient |
Capnograph | |
---|---|---|
Immediately after intubation, CO2 absent or very minimal |
• Inadvertent esophageal intubation |
• Calibration error
• Disconnection of the CO2 analyzer or the sample tube |
Exhaled CO2 present, then suddenly absent |
• Accidental tracheal extubation • Disconnection of breathing circuit • Apneic spells • Cardiac arrest• Severe bronchospasm • Complete obstruction of ETT (e.g., mucous plugging or kinking) |
• Disconnection of the CO2 analyzer or the sample tube
• Water condensation or secretions the CO2 analyzer or in sampling tube
• Failure of the capnograph |
12.2.2.3.1 The Inspiratory Baseline (Phase I)
Inspired gas should be void of carbon dioxide. Thus, the displayed CO2 level should be zero; otherwise, there must be either rebreathing of CO2 from the patient due to high apparatus dead space or the administration of exogenous carbon dioxide. Especially in very small infants, CO2 rebreathing can occur if the tidal volume is relatively low compared to the apparatus dead space. A leak around the endotracheal tube may reduce CO2 rebreathing (Claure et al. 2003); however, it may dampen both tidal volume and P etCO2 measurements considerably (Schmalisch et al. 2012).
12.2.2.3.2 The Expiratory CO2 Increase (Phase II)
During expiration, the first gas is eliminated from the CO2-free anatomic dead space. Subsequently, in healthy lungs, the CO2 curve rises with a steep upward slope. Phase II can be prolonged when the delivery of CO2 from the lung is delayed, for example, due to pulmonary inhomogeneities, high resistances of the small airways, and mechanical obstructions, such as a blocked or kinked ETT. A prolonged phase II can also be caused by technical problems related to the capnograph. Technical limitations are most commonly seen in patients with stiff lungs and a low respiratory time constant when the response time of the capnograph is inadequate to follow the fast expiration.
12.2.2.3.3 The Alveolar Plateau (Phase III)
In adults and older infants, the shape of the alveolar plateau is one of the most interesting portions of the capnogram because the steepness of the slope is a function of morphometric structure and respiratory mechanics (Neufeld et al. 1992; Schwardt et al. 1991). Early phase III contains gas from the well-ventilated, low-resistance regions of the lung. Later in phase III, gas from poorly ventilated, high-resistance regions is exhaled which causes a slope of the alveolar plateau. Thus, the steepness of the alveolar plateau is commonly used as an indicator for inhomogeneities in the alveolar time constants and
ratio (Ream et al. 1995). A flat phase III indicates relatively homogeneous exhalation from airways throughout the lung. It should be noted in very small infants that phase III should be interpreted with caution as the plateau (if it exists at all) is often small, and steepness is also a function of lung growth (Ream et al. 1995). Therefore, the diagnostic value of a phase III analysis in the small neonatal population may be limited. Nevertheless, the appearance of phase III in the capnogram indicates that alveolar gas has been sampled.

12.2.2.3.4 The Decrease of Exhaled CO2 at the Beginning of Inspiration (Phase IV)
After expiration, the fresh gas from the breathing circuit rinses out the carbon dioxide from the previous exhalation. Thus, there should be a quick decrease of the end-expiratory CO2 at the initiation of inspiration. Several techniques (e.g., tracheal gas insufflation) (Davies and Woodgate 2002; Miller et al. 2004) have been developed to reduce rebreathing and dead space ventilation such that the tidal volume is more efficiently used for gas exchange. However, for various reasons, these techniques have seen limited application in the neonatal and pediatric populations. A delayed decrease of the expired CO2 may be caused by a respiratory circuit with low flow, such that CO2 may accumulate in the inspiratory limb of the circuit. Furthermore, a prolonged phase IV can also be associated with technical failures of the capnograph (e.g., a slow response time of the CO2 analyzer).
12.2.2.4 Relationship Between P etCO2 and P aCO2
In infants with normal pulmonary function and well-matched
, the P etCO2 can provide an accurate estimate of the PCO2 in arterial blood (P aCO2) (Wu et al. 2003; McDonald et al. 2002; Bhat and Abhishek 2008), for all patient populations from the extremely low-birth-weight infant (Amuchou and Singhal 2006) through adults (McSwain et al. 2010; Wu et al. 2003; McDonald et al. 2002; Bhat and Abhishek 2008; Amuchou and Singhal 2006). Noninvasive measurement of P etCO2 clearly allows for an accurate estimation of P aCO2 in patients with minimal dead space ventilation without the inherent need for the time delay or the blood removal associated with performing an arterial blood gas analysis. The relationship between P etCO2 and P aCO2 in patients with elevated dead space is discussed in more detail below.

P etCO2 monitoring has several advantages for all patients requiring intensive care, from preterm newborns through elderly adults (McDonald et al. 2002; Rozycki et al. 1998), which include:
-
Decreased blood loss by arterial sampling for blood gas analysis
-
Lower risk of infection related to a decreased number of central venous line or arterial line entries
-
Decreased costs
-
Availability of continuous data
However, as shown in Table 12.6, several physiologic and technical factors as well as disease states can affect P etCO2 measurements and potentially make it less reliable as a surrogate for blood gas interpretation.
The lack of consistency in the agreement between P etCO2 and P aCO2 has led to significant controversy in the medical literature with regard to the usefulness of P etCO2 measurements in ventilated patients, especially in the neonatal population. McDonald et al. (2002) and Wu et al. (2003) have shown in large clinical studies in critically ill mechanically ventilated infants that P etCO2 correlates with P aCO2 and provides a clinically relevant, reliable estimation of ventilation for most infants. Similar results were found by Rozycki et al. (1998) investigating 45 newborn infants receiving mechanical ventilation. They suggest that capnography in these patients may be useful for trending or screening patients for abnormal arterial CO2 values. In contrast, Tobias et al. (Tobias and Meyer 1997a) found in intubated infants that P etCO2 does not accurately predict P aCO2 and that transcutaneous carbon dioxide (P tcCO2) measurements are more accurate. To the same result came Tingay et al. (2005) during neonatal transport as there was an unacceptable under-recording of the P aCO2 likely due to the technical limitations of the sidestream technology used.
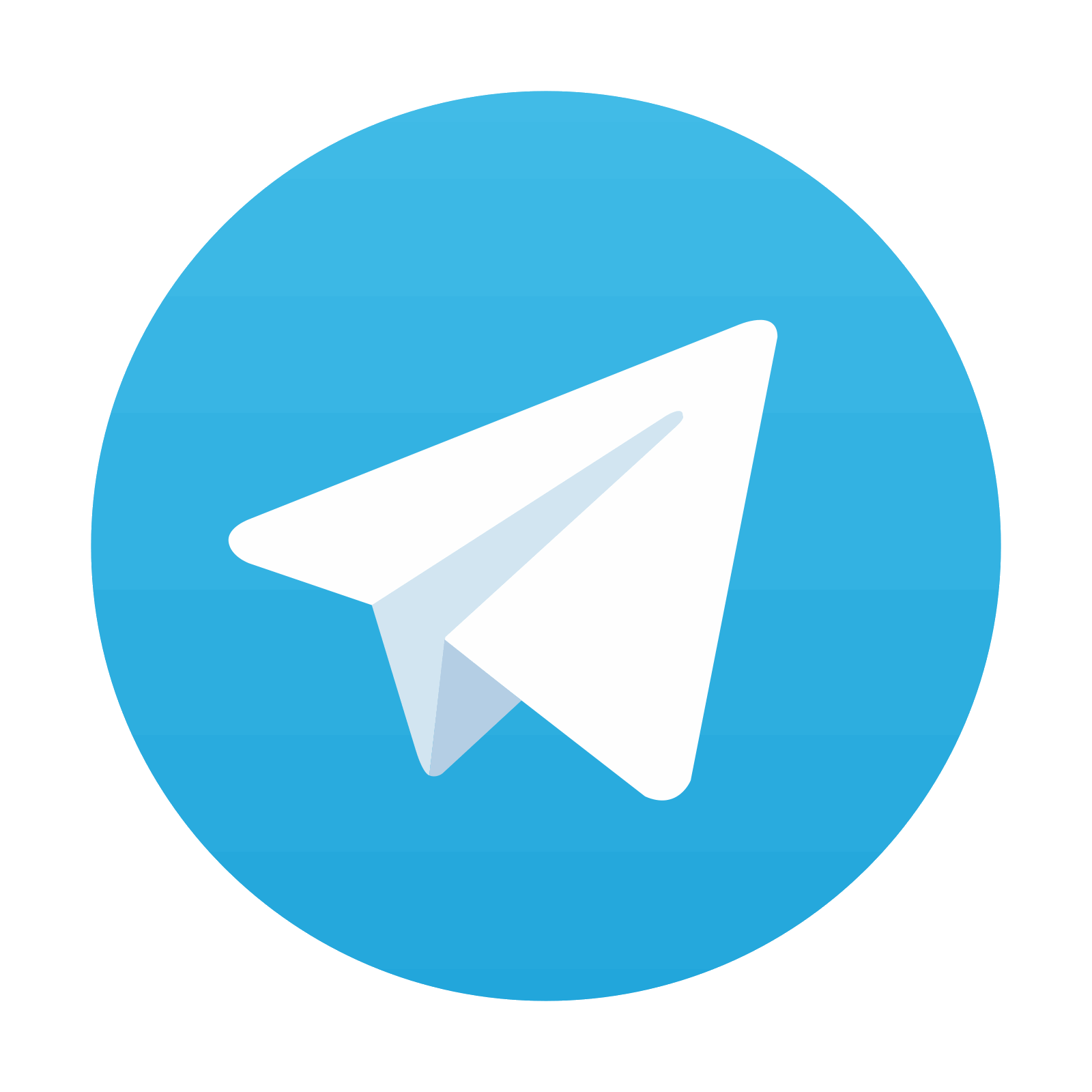
Stay updated, free articles. Join our Telegram channel
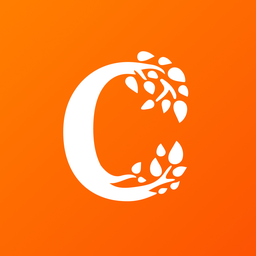
Full access? Get Clinical Tree
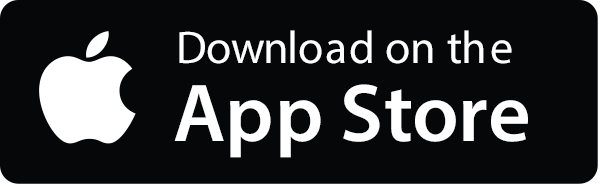
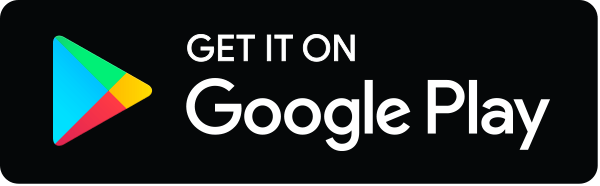