Abstract
Every cell in an individual has a unique chromosome complement, with 20 000–25 000 genes coded into a DNA sequence of 3 billion base pairs, packed into 23 pairs of chromosomes: a total of 46 chromosomes in each diploid human cell. All of these cells have the same genetic information, copied during mitotic divisions by replicating the DNA during each cell cycle. The pattern of gene activity in each cell (gene expression/transcription) dictates its function and fate, enabling different cells to differentiate and carry out distinct functions.
Stem Cells and Stem Cell Lines
Every cell in an individual has a unique chromosome complement, with 20 000–25 000 genes coded into a DNA sequence of 3 billion base pairs, packed into 23 pairs of chromosomes: a total of 46 chromosomes in each diploid human cell. All of these cells have the same genetic information, copied during mitotic divisions by replicating the DNA during each cell cycle. The pattern of gene activity in each cell (gene expression/transcription) dictates its function and fate, enabling different cells to differentiate and carry out distinct functions.
After an oocyte has been fertilized, the one-cell zygote is totipotent, with the potential to give rise to a complete organism, including both embryonic and extraembryonic cells. As cell division proceeds, blastomeres lose the potential to give rise to an entire organism, and by the time that a fully expanded blastocyst has formed, three types of morphologically and molecularly distinct cells have emerged: trophectoderm cells surround an inner cell mass, which contains epiblast progenitor cells and primitive endoderm cells. The embryo itself is derived exclusively from epiblast progenitor cells; trophectoderm cells will form fetal components of the placenta, and primitive endoderm will form the yolk sac, which is derived from extraembryonic endoderm. Continued development of the embryo requires the support of all of these extraembryonic cell types.
Epiblast progenitor cells of the blastocyst inner cell mass are considered to be pluripotent, as they have the potential to give rise to the three primary germ layers that will form all of the tissues of the fetus: mesoderm, endoderm and ectoderm. Significantly, these progenitor cells do not have the potential to give rise to a whole organism without the supporting extraembryonic cells. Following implantation, the pluripotent embryonic cells become committed to more specialized cells that increasingly lose their potential to contribute to all three germ layers. Multipotent cells have been identified in the developing embryo and in the adult, which have the potential to continually give rise to the same cell (self-renew) and also have the potential to give rise to other cells with a more specialized function. Hematopoietic stem cells are multipotent cells that replenish all blood cells by dividing to form two types of cell: one daughter cell maintains a stem cell population, and the second daughter cell has the potential to continue to alter its pattern of gene expression and differentiate. The daughter cells that become more specialized with each cell division go through distinct populations of transit amplifying cells, proliferative cells that retain their self-renewal property until they reach the end of their production line and are terminally differentiated. Other types of stem cell, such as neural stem cells, are oligopotent, giving rise to diverse but restricted populations of specific self-renewing subtypes. Unipotent cells, such as spermatogonial stem cells, are self-renewing cells that have the potential to give rise to a single lineage, spermatogonia. Figure 7.1 illustrates a hierarchy of stem cell potential.
Two properties are unique to all types of stem cell:
1. They have the capacity for long-term self-renewal.
2. They have the potential to give rise to cells other than themselves.
A stem cell line is a population of cells that has been grown and maintained in vitro. When maintained under appropriate conditions, these cells continue to grow in tissue culture for very long periods of time.
Figure 7.1 Potential pathways for stem cell development.
Mammalian Stem Cell Lines
Following the derivation and culture of human embryonic stem cell lines in the late 1990s (Thomson et al., 1998), debate and controversy escalated surrounding the use of surplus human embryos donated for research. Despite the controversial ethico-legal perspectives, in many countries throughout the world IVF clinics have embryos that are either unsuitable for treatment or are surplus to the patient’s requirements. Given the opportunity for appropriate counseling and informed consent, many patients choose to make a contribution to science by donating surplus embryos for research rather than allowing them to perish (Franklin et al., 2008). There is no doubt that embryos donated for stem cell research represent a very valuable resource for scientific investigation, with the potential to make a significant contribution to our understanding of early developmental processes and the molecular pathology of disease. Stem cell biology has become an integral part of ART; the principles and the science underpinning this new area of developmental and regenerative biology should be viewed within the perspective and frame of reference of the first stages of postimplantation development, as presented in the brief synopsis in Chapter 6.
Human Embryonic Stem Cells (hESCs)
Human embryonic stem cells are derived from the in-vitro expansion of epiblast progenitors within the inner cell mass of a preimplantation blastocyst. hESCs are capable of indefinite self-renewal, and maintain the potential to differentiate into cell types from the three embryonic germ layers (pluripotency). Because they remain pluripotent when maintained in vitro, they provide an ideal resource for investigating and studying the pathways that lead to the establishment of cells that might be relevant to clinical treatment, such as dopamine-producing neurons and insulin-producing cells of the pancreas.
The goal of hESC research is to elucidate the pathways that direct differentiation in vitro, with the aim of providing functional and therapeutically relevant cells. These cells can be used to investigate mechanisms of disease progression, and for research into drugs that might inhibit or reverse pathological processes. hESCs also provide an insight into aspects of early embryonic development that are otherwise inaccessible to research, due to ethical and practical considerations; they can be used as a tool to investigate how cells can be manipulated to regenerate damaged or diseased cells in the human body. Lastly, hESC-differentiated cells may eventually prove to be useful in cell transplantation approaches for the treatment of disease (see Trounson & DeWitt [2016] and Chen et al. [2014], for reviews).
Several strategies have been applied to promote the differentiation or selection of therapeutically relevant cell types, including the addition of growth factors or cytokines, as well as other ways of manipulating gene expression. One major hurdle to this approach is that the process of directing the differentiation of hESCs into functionally relevant specialized cells is very inefficient, and their intrinsic potential can allow them to switch from pluripotency toward uncontrolled differentiation during expansion in culture. This inefficiency may be the result of heterogeneity within a starting stem cell population, in addition to the lack of information about the developmental events that promote the emergence of specialized cells in vivo – differentiation into a particular lineage is a highly complex process, controlled by a multitude of overlapping parameters. The constraints of in-vitro culture conditions also hinder the ability to mimic in-vivo events.
The large-scale availability of treatments involving pluripotent stem cells will require further research to elucidate the fine details of the growth and stability of the cells, as well as a secure and thorough regulatory pathway to ensure their safety.
Tissue stem cells in fetal or adult tissue can supply cells to parts of the body that require a continuous supply of regenerative cells. Tissue stem cells include blood and intestinal stem cells; these are partially committed cells that can function to regenerate their respective adult tissues as required. They commonly reside in a microenvironment (niche) in a quiescent state where they are provided with signals and support that promote the maintenance of self-renewal. Stem cells exit the niche as they undergo cellular commitment/differentiation.
Multipotent Stem Cells
All blood cell types are continuously replaced from a store of hematopoietic stem cells (HSCs) in the bone marrow.
The lining of the gut (gut epithelium) has intestinal stem cells in the small intestine that produce four different cell lineages (Paneth, goblet, absorptive columnar, enteroendocrine).
Epidermal stem cells in the skin and hair follicle can regenerate damaged epithelium.
Skeletal muscle stem cells (satellite cells) are quiescent cells that can also give rise to committed progeny such as myofibers in response to injury or disease.
Oligopotent Stem Cells
Neural stem cells are restricted self-renewing subtypes that give rise to three lineages: neurons, oligodendrocytes, astrocytes.
Unipotent Stem Cells
Spermatogonial stem cells give rise to spermatogonia.
Leukemias, lymphomas and other blood disorders have been successfully treated with bone marrow transplants since the 1960s. The donor tissue must be human leukocyte antigen (HLA) matched to that of the recipient, or the cells will be rejected by the recipient’s immune system.
During the past decade, IVF in combination with preimplantation genetic diagnosis (PGD) has been used to select embryos that are HLA matched to a sibling who has a blood disorder. Cord blood isolated from the baby (‘savior sibling’) at the time of delivery is then prepared to provide a supply of HSCs for transplant to the sibling.
It had been suggested that HSCs may have ‘plasticity,’ i.e., the ability to engraft in other locations and then transdifferentiate to cell types appropriate to their new location. However, so far this concept is unsubstantiated, and there is no evidence that blood-forming stem cells can serve as a significant source of regenerative cells in the repair of nonblood tissues.
Differentiation
Cells can be distinguished from one another by their patterns of gene expression, which includes expression of both protein coding and noncoding RNAs, the secretion of proteins, their response to extracellular signals and the distribution of epigenetic chromatin modification. Changes to any or all of these processes can influence the differentiation of stem cells. For example, hESCs express the transcription factors OCT4, NANOG and SOX2 and require extracellular signals such as fibroblast growth factor (FGF) and Activin/Nodal to maintain self-renewal. Changes in the levels and balance between FGF and Activin/Nodal can influence the maintenance and differentiation of hESCs: in the presence of too much Activin/Nodal they resemble endoderm cells, and too little Activin/Nodal will cause differentiation into ectoderm cells. The mechanisms involved in the maintenance of hESCs is poorly elucidated, but is likely to involve cell signaling pathways that function at different levels, with multiple feedback controls and intercellular gene regulation:
1. Gene expression: transcription factors are proteins that function to promote or repress gene expression at the DNA level. For example, OCT4 is thought to maintain hESCs by binding to genomic regulatory regions to promote the expression of pluripotency-associated genes and repress the expression of genes associated with differentiation.
2. Extracellular signals: small proteins that are produced and secreted (cytokines) by a stem cell niche can contribute to the maintenance of self-renewal. These proteins are often required to maintain stem cells in culture, such as the addition of cytokines FGF and epidermal growth factor (EGF) to neural stem cells grown in vitro.
3. Chromatin modifications: DNA is packaged with histones to form nucleosomes. Post-translational modification of histone tails forms a code that can determine states of gene expression that are heritable, modifying gene expression by influencing the access of transcription factors to genomic regulatory regions.
4. Physical context: the presence of extracellular matrix and physical contact with other cells can influence the maintenance and differentiation potential of stem cells.
From Trounson & DeWitt (2016).
Mesenchymal stem cells (MSCs) from bone marrow, placenta, umbilical cord and adipose tissue are being used in clinical trials for bone repair, joint and lower back pain, and graft versus host disease (GvHD).
MSCs have been applied as therapy for myocardial infarction, cartilage repair, diabetes, and pulmonary, neurological and liver disease.
Neural stem cells from adult and fetal tissues are being studied for diseases of the eye, spinal cord injury and neurodegenerative disorders such as Parkinson’s disease and amyotrophic lateral sclerosis.
Limbal stem cells of the cornea have been approved for autologous therapies and as allogeneic transplants involving donor cells.
Gene therapy strategies are being studied as a means of DNA-editing hematopoietic stem cells in order to replace a mutated gene with a normal copy to cure single-gene diseases such as thalassemia, sickle cell disease, adrenoleukodystrophy and severe combined immunodeficiency.
Potential Therapeutic Applications for ESCs
1. Platform for drug discovery: hESCs can be differentiated into clinically relevant cells from individuals that harbor disease-associated gene expression. For example, hESCs have been established from individuals with amyotrophic lateral sclerosis, and these hESCs were differentiated to motor neurons, the cells that are damaged in these patients. These hES-differentiated cells can be used to screen for small molecules (drugs) that might inhibit the progression of the disease and be effective in patient treatment.
2. Exogenous: transplantation of stem cells that have been differentiated in vitro toward a particular cell lineage, e.g., neuronal stem cells for neurodegenerative diseases that involve death or dysfunction of just one, or a few, cell types, such as dopaminergic neurons to treat Parkinson’s disease, insulin-producing beta-cells to treat diabetes, etc. This approach has at least two significant considerations:
in-vitro stem cell differentiation must be completely controlled, as there may be a possibility of malignant transformation
transplant rejection unless the cells are immune matched.
3. Autologous: the patient’s own stem cells are manipulated in vitro to induce/repress gene expression and returned to the specific site that requires healing/treatment.
4. Endogenous stem cell renewal: the patient’s own stem cells are manipulated to renew themselves in situ; this requires:
identification of stem cells in different parts of the body
interpretation of how they interact with their niche/microenvironment.
Evolution of Stem Cell Research
The derivation of human ESCs in 1998 ignited an explosion of interest in stem cell biology and its therapeutic potential, and research in the field evolved very rapidly over the subsequent decade. In the words of the French philosopher Auguste Comte (1798–1857) ‘To understand science, it is necessary to know its history,’ and this is particularly true of stem cell science, where each significant achievement has been based upon the findings of prior decades of research. Successful derivation of human ESCs was based on research using murine ESCs, and this required information that was gained from previous stem cell models using mouse and human embryonal carcinoma cell lines. Similarly, the recent elucidation of discrete factors that can induce somatic cells into forming pluripotent stem cells (iPSCs) depended on studies in mouse and human embryonic stem cells. There is no doubt that novel trends will continue to emerge, and in order to fully comprehend the potential and the possible directions that lie in the future of stem cell research, it is important to be aware of the milestones that have marked its evolution so far.
Early Culture Systems
During the early 1960s, Robert Edwards recognized the extraordinary potential of stem cell biology, and in collaboration with Robin Cole and John Paul in Glasgow, he isolated stem cells from the inner cell mass (ICM) of rabbit blastocysts. They cultured zona-free rabbit ICM on collagen surfaces, sometimes with HeLa feeder layers, and established cell colonies that showed differentiation to muscle, blood islands, neurons and complex groups of differentiating cells (Figure 7.2). Four immortal cell lines (two epithelioid and two fibroblastic) that survived more than 200 generations of subculture were established and cryopreserved. All cell lines remained diploid for several generations (Cole et al., 1965, 1966; Edwards, 2008).
(a) Cell masses derived from a zona-free rabbit blastocyst.
(b) Muscle differentiating after continued culture.
(c) A single blood island that developed among cell outgrowths from rabbit ICM.
(d) A group of neurons in the same outgrowths.
(e) A complex group of differentiating cells in which muscle cells are mixed with various types of differentiating cells – a pathologist discerned several cell types in this mass of cells.
Figure 7.2 Cell colonies derived from intact zona-free rabbit blastocysts after 20 days in culture (Cole et al., 1966).
In further studies by Richard Gardner (1968), ICM cells isolated from mice with marker (coat color) genes were injected directly into the blastocoelic cavity of recipient mice, forming chimeras which showed that the grafted cells had colonized several different tissues; this indicated that the injected ICM cells were multipotent. After human blastocysts first became available through IVF, Edwards and his team attempted to prepare human ESCs in vitro (Fishel et al., 1984), but this early work had to be abandoned due to ethico-legal problems surrounding the use of human embryos for research.
Embryonal Carcinoma (EC) and Embryonal Germ (EG) Cell Lines
In the early 1970s, stem cell lines that could be propagated in vitro were derived from murine teratocarcinomas (Kahn and Ephrussi, 1970); these cell lines were capable of unlimited self-renewal and multilineage differentiation. Mouse EC cells express antigens and proteins that are similar to cells present in the ICM, which led to the concept that EC cells are an in-vitro counterpart of the pluripotent cells present in the ICM (Martin, 1981); this provided the intellectual framework for working toward derivation of both mouse and human embryonic stem (ES) cells. Human EC lines were established in 1977 (Hogan et al., 1977), and these proved to differ from mouse EC lines, both in expression of surface markers, and in their in-vitro properties. Unlike mouse EC lines, the human cells are highly aneuploid and have a limited ability to differentiate into a wider range of somatic cell types. Nonetheless, these human cell lines provided a useful model in which to study cell differentiation (Andrews et al., 1984b; Thompson et al., 1984; Pera et al., 1989). Both mouse and human EC culture systems enabled numerous improvements in technique and methodology, as well as initiating studies on factors that are involved in the control of differentiation in vitro.
Stem cell lines derived from mouse testicular teratomas (EG cells) were found to contribute to a variety of tissues in chimeras, including germ cells (Stevens, 1967); this provided a practical way to introduce modifications to the mouse germline (Bradley et al., 1984). Pluripotent EG cells were successfully derived directly from primordial germ cells in vitro in 1992 (Matsui et al., 1992; Resnick et al., 1992).
Mouse Embryonic Stem Cells
Using culture conditions that had been used for mouse EC cells (inactivated fibroblast feeder layers and serum), the first mouse ES cell lines were derived from the ICM of mouse blastocysts (Evans and Kaufman, 1981; Martin, 1981). It was subsequently found that the efficiency of mouse ES cell derivation is strongly influenced by genetic background and that different culture conditions were required for strains that were initially thought to be nonpermissive. Further experiments revealed that mouse ES cells could be sustained by conditioned medium harvested from feeder layers, in the absence of the feeder cells themselves. Fractionation of conditioned medium led to the identification of leukemia inhibitory factor (LIF) as one of the cytokines that sustains mouse ES cells (Smith et al., 1988; Williams et al., 1988). LIF activates the transcription factor Stat3, which inhibits differentiation and promotes viability. Further investigation of the signaling pathways showed that the proliferative effect of LIF requires a finely tuned balance between positive and negative effectors/factors. If serum is removed from the medium, mouse ES cells can be maintained in an undifferentiated state by adding LIF in combination with bone morphogenetic protein (BMP), a member of the TGF-alpha superfamily (Ying et al., 2003).
The subsequent two decades of research using murine ESCs led to numerous advances in culture system techniques and technology, and the identification of several types of in-vitro differentiated cells (including neural tissue) introduced the therapeutic potential and hope of eventual therapies to treat degenerative diseases – neurodegenerative disease in particular. The murine ES model contributed enormously to many different aspects of developmental biology: a large collection of genes, factors, markers and signaling pathways involved in differentiation have now been identified, providing clues toward achieving directed differentiation of these pluripotent cells in vitro (Yu et al., 2007).
Human Embryonic Stem Cells
A considerable delay (17 years) ensued between the derivation of mouse ES cells in 1981 and the first establishment of human ES cell lines in 1998. This was due to at least two factors:
1. Suboptimal media and conditions for human embryo culture meant that human blastocysts were rarely available (especially for research purposes). Media optimized for extended culture were introduced during the mid-1990s, and blastocyst culture then became a more practical reality.
2. Initial culture systems for hESC derivation were based on those that were successful in the mouse, and it eventually became apparent that there are significant species-specific differences between mouse and human ES cells.
Isolation of ICMs from human blastocysts was reported in 1994 (Bongso et al., 1994), but they were cultured in conditions that allowed derivation of mouse ES cells, and this resulted only in differentiation of the human cells instead of their derivation into stable pluripotent cell lines. In the mid-1990s, ES cell lines were derived from two nonhuman primates: the rhesus monkey and the common marmoset (Thomson et al., 1995, 1996). Using experience gained from these primate models, in 1998 Thompson and his colleagues then reported the isolation of pluripotent stem cell lines derived from human blastocyst ICM cultured on inactivated mouse feeder layers. These cell lines expressed markers for pluripotency and could be maintained undifferentiated in long-term culture. The cultured cells also maintained the potential to form derivatives from all three germ layers when injected into severe combined immunodeficiency (SCID) mice. Media containing LIF and its related cytokines, required for mouse ESCs, failed to support human or nonhuman primate ES cells (Thomson et al., 1998; Dahéron et al., 2004; Humphrey et al., 2004); the fact that fibroblast feeder layers supported both mouse and human ES cells was apparently a fortunate coincidence.
Reubinoff et al. (2000) then reported directed differentiation of hESCs, producing three neural cell lines (astrocytes, dendrocytes, mature neurons) from an early neural progenitor stem cell in spontaneously differentiating cultures. A huge expansion in research activity followed this initial report, and over the next few years panels of markers associated with pluripotency and with stages of differentiation were identified. Research continues into elucidating pathways of differentiation and identifying factors that sustain hESCs in culture, as well as active factors that decay with de-differentiation, or change during re-differentiation. The body of published literature surrounding hESCs is now vast; by manipulating culture conditions, spontaneous differentiation to numerous different cell types has been observed, including beating heart cells (Mummery et al., 2002), insulin-secreting cells, hepatocytes, cartilage, etc. Protocols are now available for directing at least partial differentiation of hESCs toward numerous different fates: early endoderm, hepatic cells, pancreatic cells, cardiomyocytes, endothelial cells, osteogenic cells, hematopoietic cells, lymphocytes, myeloid cells, etc. (see Sullivan et al., 2007). hESC lines have also been genetically modified, with fluorescent reporter genes introduced into key gene loci that can be traced during in-vitro differentiation in order to identify subsets of cells along developmental pathways (Davis et al., 2008a; Hatzistavrou et al., 2009).
Somatic Cell Nuclear Transfer: Cloning
See (Figure 7.3).
1. Therapeutic cloning: the nucleus of an adult somatic (differentiated) cell is reprogrammed by insertion into an enucleated donor oocyte. The oocyte is then activated by electrofusion to stimulate cleavage, grown in culture to the blastocyst stage, and the ICM of this blastocyst used for stem cell derivation. The resulting stem cell lines are immunologically identical (HLA matched) to the somatic cell that was reprogrammed and could theoretically be used for potential therapies without transplant rejection. The technique has been successful in nonhuman primates (rhesus macaque), although with very low efficiency: two primate ESC lines were derived from the use of 304 oocytes (Byrne et al., 2007). A report that several patient-specific stem cell lines had been established in Korea subsequently proved to be based on data that was fabricated, and the papers were withdrawn (Hwang et al., 2004, 2005). In view of the extreme difficulty in obtaining donor oocytes, and the inefficiency of the technique so far, it may be that pursuing research with iPS cells is a better strategy than therapeutic cloning.
2. Reproductive cloning: somatic cell nuclear transfer (SCNT) is carried out as for therapeutic cloning, but the resulting embryo is transferred to the uterus. Dolly, the much-celebrated sheep, was the first example of successful mammalian reproductive cloning, demonstrating that a differentiated adult cell could be reprogrammed to generate an entire organism (Wilmut et al., 1997). This success confirmed John Gurdon’s remarkable discoveries during the 1950s, when he was able to produce tadpoles after somatic cell nuclear transfer into Xenopus laevis eggs (Gurdon, 1962). The technique has been used in a variety of large and small animals, including cows, goats, mice, pigs, cats, rabbits and a gaur. However, reproductive cloning is both expensive and highly inefficient; more than 90% of cloning attempts fail, and imprinting defects have been identified in cloned animals, with abnormalities of immune function and growth and numerous other disorders. Experiments with cloned mice indicate that approximately 4% of their genes function abnormally, due to imprinting defects.
Figure 7.3 Transfer of a diploid somatic cell nucleus into an enucleated metaphase II oocyte followed by activation by electrofusion leads to the creation of an embryo that has the chromosome complement of the somatic cell. A resulting blastocyst stage embryo can be transferred to a recipient uterus and allowed to develop to term into an animal with characteristics of the transferred somatic nucleus (reproductive cloning), or inner cell mass cells may be used to generate stem cell lines that are genetically matched to the donor of the somatic cell (therapeutic cloning).
Epiblast Stem Cells (EpiSCs)
As described in Chapter 6, one of the first stages of postimplantation development is the separation of the ICM into two lineages, the hypoblast and the pluripotent epiblast.
Pluripotency can be considered as two states: newly segregated epiblast cells contain ‘naïve’ or ground state pluripotency, and following implantation these respond to signals from the extraembryonic tissues to become primed for differentiation. The two types of cells are characterized by expression of core pluripotency factors Oct4, Sox3 and Nanog, but they differ in their expression of other genes and their culture requirements.
Experiments with mouse embryos established that the epiblast in the late blastocyst is functionally and molecularly distinct from blastomeres, and from the ICM (Nichols and Smith, 2009). A strategically important milestone was reached in 2007, with the isolation of primed pluripotent stem cell lines derived from postimplantation (E5.5 to E6) mouse and rat epiblast (Brons et al., 2007; Tesar et al., 2007). Intriguingly, these EpiSCs differ significantly from mouse ESCs, but have key features in common with human cells. The two factors required for mouse ESC derivation (LIF and/or BMP4) have no effect on epiblast cell isolation, a similar situation to human ESC derivation. Instead, the signaling factors that are important for human ESCs (FGF and Activin/Nodal) are apparently critical for EpiSC derivation. The pattern of gene expression by EpiSCs differs from that of mouse ESCs, but they do retain the ability to proliferate indefinitely, as well as the potential for multilineage differentiation. Mouse ES cells can be induced to become EpiSCs, and the reverse transition has also been observed (Bao et al., 2009). It is possible that the unique properties that hESCs have in common with EpiSCs could reflect a different origin that had not previously been recognized in hESCs; i.e., they may represent a later stage of development (postimplantation epiblast) than mouse ES cells. Activin/Nodal signaling seems to have an evolutionarily conserved role in the maintenance of pluripotency. This also reinforces the significant species-specific differences in genetic and signaling pathways for lineage specification between humans and rodents. Several key TGF-ß signaling pathways were found to be enriched and differentially expressed in the human EPI and TE, and inhibiting this pathway downregulated Nanog expression in human, but not in mouse EPI cells – TGF-ß signaling is required for the development of pluripotent EPI in human blastocysts (Roode et al., 2012). The differences between species in expression of early lineage-specific genes have significant implications for developmental control and stem cell derivation.
Induced Pluripotent Cell Lines (IPSCs/PiPSCs)
The concept of reversing the programming of differentiated tissues to pluripotent states was introduced with the first somatic cell nuclear transfer (SCNT) experiments by John Gurdon during the late 1950s, using Xenopus oocytes (Gurdon, 1962). Several decades later, the birth of ‘Dolly the Sheep’ provided the first confirmation in mammals that a differentiated somatic cell could be converted to a totipotent state by inserting its nucleus into an enucleated oocyte. Dolly was born at the Roslin Institute in Edinburgh on July 5, 1996. The somatic cell nucleus was taken from a mammary gland cell and transferred into an unfertilized ovine oocyte that was then stimulated to divide by electrical pulse activation; the hybrid cell developed to blastocyst stage and was transferred to a recipient surrogate mother. Dolly died on February 14, 2003 from a progressive lung disease, 5 months before her 7th birthday.
With accumulated information regarding pluripotency in ESC, pinpointing key genes that might be used to reprogram somatic cells became a major research goal. Panels of genes that are enriched in ESC populations and thought to be involved in maintenance of pluripotency were screened, with the target of identifying factors that have the ability to reprogram somatic mouse cells into proliferation. From a panel of 24 target genes, four factors were found that together induced a transformation of mouse fibroblasts into cells that closely resemble mouse ES cells: OCT4, SOX2, c-Myc and Klf4 (Takahashi and Yamanaka, 2006). The experiments were conducted by using retroviruses to insert key pluripotency genes into the fibroblasts; the cells that resulted had properties analogous to ESCs in culture and also formed teratomas when injected into mice. The same technique was subsequently applied to successfully reprogram human fibroblasts into hES-like cells (Takahashi et al., 2007a; Lowry et al., 2008). A further independent study using human cells screened a panel of 14 genes that are enriched in hESCs and succeeded in reprogramming human fibroblasts with the introduction of genes for OCT4, SOX2, NANOG and LIN28 (Yu et al., 2007). Human iPS cells satisfy all the original criteria proposed for characterization of hESCs: morphology is similar, they express typical hESC surface antigens and genes, differentiate into multiple lineages in vitro, and when injected into SCID mice they form teratomas containing cells derived from all three primary germ layers. Since this initial report, numerous combinations of somatic cell type and cocktails of factors have been studied and continue to be investigated: some cell types can be reprogrammed more efficiently than others, and different types of cell respond to different expression levels and combinations of factors. The number of factors investigated continues to grow, and the original four now only head a list of related proteins that seem to enhance the efficiency of transformation in some cells (Heng et al., 2010). Efforts to elucidate the mechanisms by which such a limited number of transcription factors can erase and reprogram a differentiated state continue: the DNA-binding sites of OCT4, SOX2 and NANOG have been studied, and it seems that these three factors can also activate or repress the expression of many other genes, including transcription factors that are important during early stages of development (Boyer et al., 2005).
OCT4
POU domain transcription factor.
Expression restricted to pluripotent cells of the embryo.
Expressed in undifferentiated ES, EC and EG cells.
Essential for formation of the ICM.
In the absence of OCT4, all cells in the embryo become trophectoderm.
Deletion in ES cells causes differentiation into trophectoderm.
Overexpression causes differentiation to endoderm/mesoderm.
SOX2
High mobility group (HMG) box transcription factor.
Expressed in ICM and trophectoderm.
Required for development of the embryonic lineage.
Also required for proliferation of extraembryonic ectoderm.
Interacts specifically with OCT4 to influence expression of target genes.
SOX2 controls OCT4 expression, and they perpetuate their own expression when they are co-expressed.
c-Myc
Transcription factor that activates expression of a large number of genes through binding on enhancer box sequences (E-boxes).
Has a direct role in DNA replication, can drive cell proliferation by upregulating cyclins/downregulating p21.
Also has roles in cell growth, apoptosis, differentiation, stem cell renewal.
Proto-oncogene: upregulated in many types of cancers.
Klf4: A Member of the Krüppel-like Family of Transcription Factors
Can act as a transcriptional activator or repressor, depending on promoter context and interaction with other transcription factors.
NANOG
Homeodomain transcription factor.
Expression is tightly associated with pluripotency.
Essential for early development and for reprogramming.
Required for development of the epiblast.
Protein-Induced Pluripotent Stem Cell Lines (piPSCs)
In further attempts to overcome the problem of viral vectors, modified versions of the protein products themselves have also been used. Stable iPSCs from human fibroblasts were generated by using a cell-penetrating peptide to deliver the four proteins OCT4, SOX2, Klf4 and c-Myc (Zhou et al., 2009; Cho et al., 2010). The cells produced by this DNA-vector free, direct protein transduction method have been called ‘protein-induced pluripotent stem cells,’ piPSCs. Experiments have been carried out using recombinant proteins, as well as proteins derived from cell fractions. Since the first discovery that somatic cells could be induced into pluripotency, methods and protocols have been refined, and a great deal of research is now focused toward practical application of the technology in defining pathophysiology of disease and potential drug discovery for treatment of human disease (Negoro et al., 2017). Pioneering studies have demonstrated that iPSCs derived from cells carrying monogenic disorders can mimic disease phenotypes in vitro when differentiated into cell types that are characteristic of the disease, providing a unique in-vitro human model that can be used for drug screening or further research (see Hamazaki et al., 2017 for review).
Germline Stem Cells and Implications for In-vitro Gametogenesis
Spermatogonial Stem Cells (SSC)
As described in Chapter 3, primordial germ cells differentiate into type A (A0) spermatogonia in the fetal testis, and spermatogonia in the seminiferous tubules differentiate into mature sperm after puberty. Type A spermatogonia in the basal compartment of the tubules divide by mitosis to form two cells, another parent type A cell and a second (type B) diploid cell that is irreversibly committed to entering meiosis in order to start the journey toward mature haploid sperm cell production. Spermatogenesis continues throughout the life of a normal adult male, producing millions of sperm each day. The relatively small population of self-renewing type A spermatogonia are known as spermatogonial stem cells (SSCs), and these adult tissue stem cells are essential for male fertility, balancing self-renewal with differentiating divisions for continuous sperm production. hSSCs are unipotent stem cells; despite stages of amplification and differentiation, they ultimately produce only one cell type: mature sperm.
Their active proliferation makes them highly susceptible to damage by chemotherapy and radiation, and therefore patients undergoing treatment for cancer may become temporarily or permanently infertile. Semen and/or testicular biopsy cryopreservation have long been offered to men prior to initiation of gonadotoxic treatment; this option is not possible for prepubertal boys, who have not yet started spermatogenesis. The potential of using SSCs as a means of preserving a patient’s fertility remains an active focus for research directed toward understanding:
1. How SSCs keep their germline identity, and their paternal-specific pattern of epigenetic imprinting
2. The testicular niche and the intercellular communications that balance self-renewal with differentiation in order to allow lifelong gametogenesis.
In 1994, Brinster and colleagues described transplantation of SSCs from fertile donor mice to the testes of infertile recipients; the recipient mice initiated donor-derived spermatogenesis and produced functional sperm (Brinster et al., 1994). Since that time, experimental SSC transplantation has been used to restore fertility, with resulting embryos or offspring, in mice, rats, goats, sheep and monkeys (Gassei & Orwig, 2016). Based upon these experiments, many centers are now cryopreserving testicular tissue or cells from prepubertal boys before they start cancer treatment, in the hope that SSC-based therapies will be available in the future. Experimental strategies under investigation to achieve in-vivo or in-vitro spermatogenesis include testicular tissue grafting, testicular tissue organ culture, SSC culture and transplantation, and iPSCs.
Testicular Tissue Grafting
Successful homotropic and heterotropic testicular tissue grafting has been reported in rodent models, with the birth of pups after production of spermatozoa capable of ICSI fertilization. Experimental xenografting of human testicular tissue in nude mice has thus far shown poor graft survival and massive germ cell loss (Del Vento et al., 2018), and although useful as an experimental model, the risk of zoonosis and epigenetic modification rules this strategy out for clinical use. Homotropic (intratesticular) grafting has the advantage that testicular tissue is transferred to its natural environment with the cells required to support spermatogenesis, the germ cell niche. Although prepubertal testicular tissue has been cryobanked in many centers over the last decade, successful clinical application by transplanting the tissue graft to restore fertility has not yet been reported. Further studies are needed to confirm the reproductive potential of spermatogonia that survive after cryopreservation, and to explore the biology of human spermatogenesis to discover whether techniques that have been successful in animal systems are likely to succeed with human tissue. Transplantation techniques will also need to be refined, with the aim of preserving the integrity of the germ cell niche that regulates self-renewal and differentiation. In the case of cancer patients, residual malignant cells may be present, and therefore this strategy may only be used for patients in whom there is no risk of malignant contamination.
Testicular Organ Culture and Three-Dimensional Cell Culture
Principles of tissue engineering have been used to achieve in-vitro spermatogenesis, aiming to provide an environment that mimics the natural extracellular environment with synthetic or biologically derived matrices in organ or three-dimensional culture. For organ culture, intact testicular tissue is layered on an agar slab suspended in medium; for three-dimensional culture, SSCs are first dissociated from their somatic cells and then suspended in a soft agar culture system, containing 50% agar. Somatic cells are included in both systems, in order to mimic the in-vivo situation and try to promote communication between the cellular compartments. Newborn mouse organ culture systems have maintained pieces of intact testicular tissue in culture, with complete spermatogenesis after 3–6 weeks of culture and production of haploid round spermatids and sperm capable of fertilization after ICSI. Although the method has not yet been reported as successful using human tissue, the mouse model may provide increased insight into the factors that drive SSC self-renewal and differentiation.
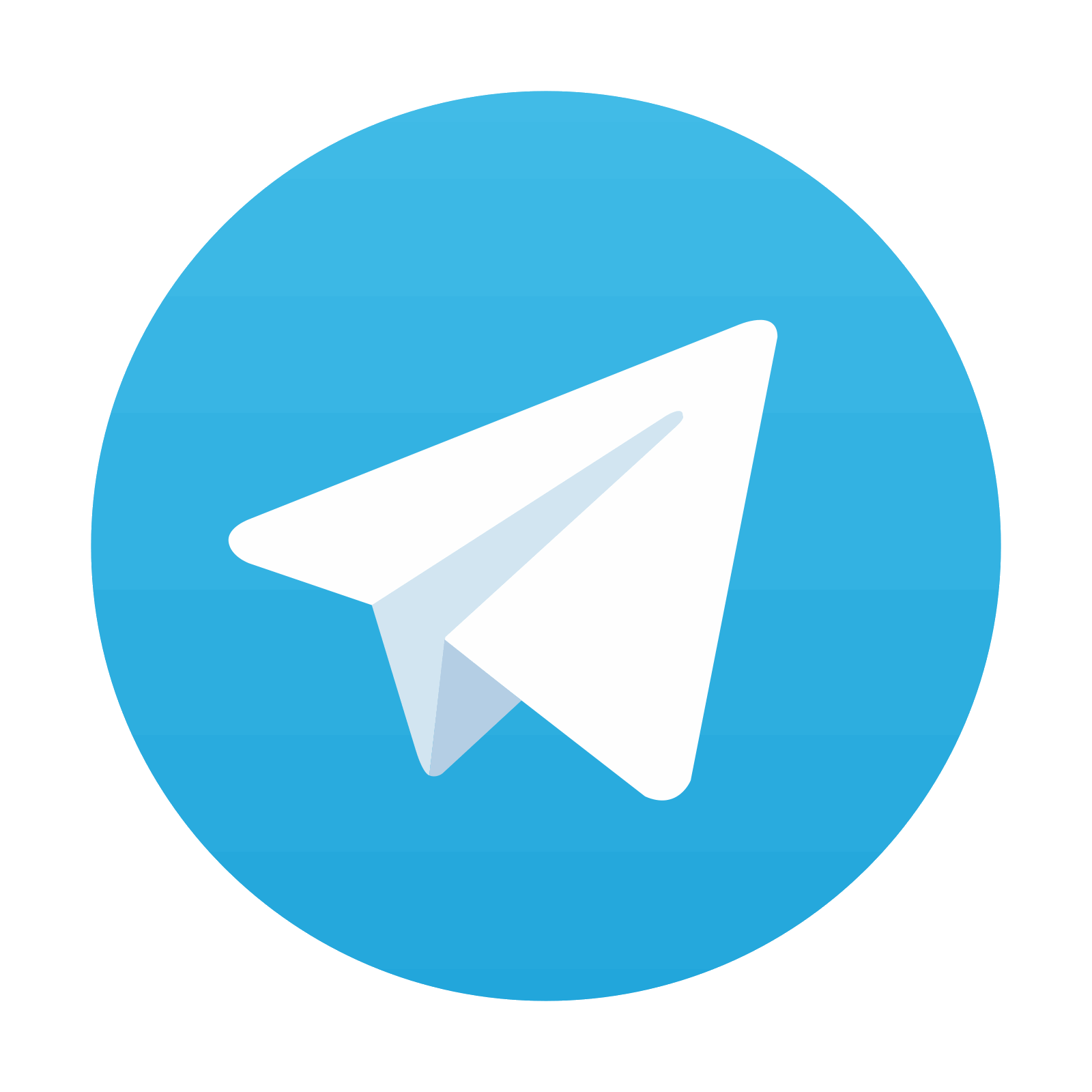
Stay updated, free articles. Join our Telegram channel
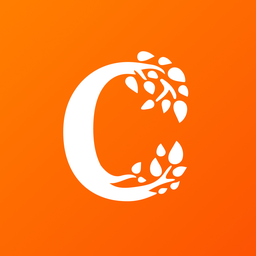
Full access? Get Clinical Tree
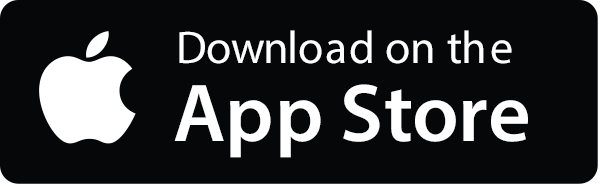
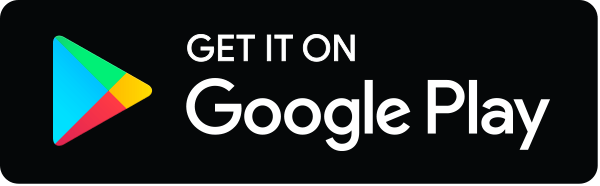
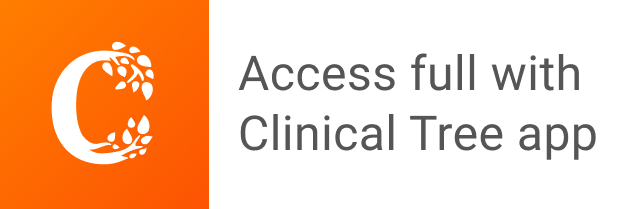