Abstract
The popularity of vitrification (literally, glass formation) as a method of cryopreservation for reproductive cells, tissues, and even organs is evident from the rising number of citations of these applications in PubMed (Figure 6.1). The success of vitrification is based on the remarkable fact that it has been possible in many cases to reconcile the extreme physical and chemical requirements of vitrification with the biological requirements for sustaining life. Essentially, the same basic physical process that produces obsidian, window panes, porcelain vases, amber, and lollipops can be applied to living cells and tissues to preserve them in a viable state for very long periods.
The basic biological feasibility of vitrification was first discovered by nature. It now appears that many “poikilohydric” animals (whose water content depends on the ambient humidity) seem to survive the winter at deep subzero temperatures in the vitreous or partially vitreous state, and this strategy of survival may be even more prevalent than freeze tolerance [1, 2]. In addition, there are complex organisms that can dry to a vitreous state and survive even at temperatures well above zero [3, 4]. Therefore, cryopreservation by vitrification is supported by a broad base of biological evidence and evolutionary experience.
Introduction
The popularity of vitrification (literally, glass formation) as a method of cryopreservation for reproductive cells, tissues, and even organs is evident from the rising number of citations of these applications in PubMed (Figure 6.1). The success of vitrification is based on the remarkable fact that it has been possible in many cases to reconcile the extreme physical and chemical requirements of vitrification with the biological requirements for sustaining life. Essentially, the same basic physical process that produces obsidian, window panes, porcelain vases, amber, and lollipops can be applied to living cells and tissues to preserve them in a viable state for very long periods.
Figure 6.1 PubMed citations related to the vitrification of reproductive cells, tissues, and organs. Curves show results of searches for “vitrification” and the stated key words, except in the case of sperm, for which references to the use of intracytoplasmic sperm injection and similarly irrelevant citations were omitted. Citations are complete through the end of 2017
The basic biological feasibility of vitrification was first discovered by nature. It now appears that many “poikilohydric” animals (whose water content depends on the ambient humidity) seem to survive the winter at deep subzero temperatures in the vitreous or partially vitreous state, and this strategy of survival may be even more prevalent than freeze tolerance [1, 2]. In addition, there are complex organisms that can dry to a vitreous state and survive even at temperatures well above zero [3, 4]. Therefore, cryopreservation by vitrification is supported by a broad base of biological evidence and evolutionary experience.
Vitrification has become popular for several reasons. It was originally proposed as a means of avoiding chemical [5, 6] or mechanical [7] damage induced by ice crystals [7–9], but it also avoids the dilemma posed by freezing whereby survival is typically an imperfect compromise between cooling too rapidly (leading to dangerous or lethal intracellular ice formation [IIF]) and cooling too slowly (leading to so-called “solution effects” injury) [10]. With vitrification, there is no optimum cooling rate, so there is no need to search for one or use controlled rate freezing devices, and survival of reproductive cells tends to be superior [11]. The virtually instantaneous vitrification and rewarming possible with reproductive cells compares favorably to the relatively lengthy and somewhat cumbersome process of slow freezing, and it is a great advantage that vitrification enables very rapid cooling without IIF, thus enabling chilling injury to be “outrun” in chilling-sensitive cells [12–15].
But vitrification has disadvantages and obstacles as well. The need for high concentrations of cryoprotectants introduces the risk of cryoprotectant toxicity and osmotic damage. Rapid cooling to liquid nitrogen temperature can induce fractures that can cut through structures such as cells or the zona pellucida [16]. Fractures can also serve as ice nucleation sites [17] that can exacerbate the problem of ice formation during rewarming [18, 19], particularly in closed container or whole organ vitrification systems. Finally, container-free vitrification methods may theoretically lead to serious sample contamination [20].
Knowledge of the principles of biological vitrification should help to overcome these and other problems. Accordingly, the purpose of this chapter is to review these principles. The reader is referred as well to earlier reviews for additional details [1, 2, 11, 21–24].
Terminology and General Orientation
It is useful to begin by defining some of the key terms that are used to describe the phenomena associated with vitrification and their relationships with one another. Considering these definitions also provides an opportunity to briefly introduce some of the central concepts related to cryopreservation by vitrification.
“Freezing” refers to the formation of ice crystals, and “thawing” refers to the melting of those ice crystals. Given that both events are nominally absent in vitrification procedures, it is inappropriate and potentially confusing to use terminology such as “freezing by vitrification” or the “melting” or “thawing” of a vitrified system, or to refer to rapid freezing methods as “vitrification” methods. Unfortunately, such misuses of terminology often occur and complicate literature searches.
“Cryopreservation” refers only to the low temperature preservation of living systems and not necessarily the means by which that preservation is achieved (i.e., freezing or vitrification). However, in practice, the term generally refers to cryopreservation by freezing unless otherwise stated. Therefore, when vitrification is the subject, referring only to “cryopreservation” can be misleading.
“Vitrification” is the solidification of a liquid into an amorphous (literally, “structure-free”) solid as a result of increasing viscosity when this is not prevented by nucleation and crystal growth [25, 26]. Vitrification takes place at the “glass transition temperature,” or TG, and is usually observed to occur when the viscosity reaches 1013 poise (1010 Pascal-seconds) [26]. This is nearly 1015 times the viscosity of plasma, suggesting a similar fold reduction in biological rates of deterioration during storage [27].
“Supercooling” is the process of cooling to below the thermodynamic freezing/melting point of a solution (TM) without ice formation. Vitrification involves supercooling by ~100 ± 30°C between TM and TG [24, 28]. Supercooling is limited by ice nucleation, which may be either catalyzed by impurities (“heterogeneous nucleation”) or induced by spontaneous rearrangements of water molecules into ice nuclei (“homogeneous nucleation”). The temperature of heterogeneous nucleation (THET) is typically stochastic, but homogeneous nucleation tends to be extremely rapid and occurs at a temperature (TH) that is therefore very well defined for more dilute solutions when cooling at less than ultrarapid rates.
The “concentration needed to vitrify” (originally abbreviated as CNV [24]) or simply CV [29] is the lowest concentration that enables vitrification at a specific cooling rate.
“Devitrification” is not the reverse of vitrification; it is the formation of ice during warming from the vitrified state [30, 31]. It often includes IIF [32, 33], which can result in damage or the death of living systems [32]. Devitrification can be detected using differential scanning calorimetry (DSC) as an exothermic event that peaks at TD, the temperature of devitrification.
“Recrystallization” is the growth of larger ice crystals at the expense of smaller ice crystals, usually during rewarming [31, 34]. When devitrification is fairly intense and results in large numbers of small crystals, the crystals that form can be too small to cause substantial damage until they recrystallize into smaller numbers of larger ice crystals that are large enough to cause lethal distortions of cellular ultrastructure [1, 35, 36]. Survival after devitrification is therefore often possible provided warming is rapid enough to “outrun” recrystallization [2]. For that reason, survival following rapid cooling and warming is not proof of successful ice avoidance.
The “critical cooling rate” is the cooling rate that is just high enough to prevent ice formation on cooling [37], and the “critical warming rate” is the warming rate that is just high enough to prevent ice formation on warming [38]. The definitions of these rates can vary depending on how much ice formation is considered insignificant. Typically, the critical cooling rate is based on preventing more than 0.2% w/w ice from forming [39]. The critical warming rate has been determined mostly for dilute solutions by extrapolating to the warming rate that is sufficient to make TD/TM equal to 0.95 [38].
The Historical Development of Ideas about Cryopreservation by Vitrification
Vitrification by Rapid Cooling
The concept of using ultrarapid cooling to induce vitrification and thereby cryopreserve living cells by preventing ice-related damage was first advanced by Stiles in 1930 [40], Luyet in 1937 [8], and Goetz and Goetz in 1938 [7, 35]. Neither Luyet nor Stiles initially presented biological evidence, but Luyet published at least six research papers on vitrification in 1938 [41], the most prominent appearing in September [42] and November [43]. Goetz and Goetz, also in November of 1938 and earlier that year, published both a cogent description of the physics of vitrification [7] and a total of five reports [35] of positive data on yeast, including one back-to-back paper with Luyet and Gehenio [43] in Biodynamica [35]. Thus, Goetz and Goetz published some of the earliest actual biological evidence in support of vitrification simultaneously with Luyet, and Stiles proposed vitrification earlier than Luyet, but it was Luyet and his colleagues who provided the first truly clear proposal and went on to publish the vast majority of work in the field for the next 20 years [23, 41]. Therefore, Luyet clearly deserves the credit he is usually given for being the original driving force behind vitrification.
In the 1930s and early 1940s, few (e.g., [44]) tools beyond rapid cooling to outrun ice crystal formation were available to pursue cryopreservation at deep subzero temperatures, which helped to sustain interest in vitrification by partial dehydration and rapid cooling. However, the evidence for vitrification was strongly questioned in 1954 [45], and by then, the alternative of colligative cryoprotection had been well established [46, 47]. Luyet issued a disclaimer restating the tentative nature of his observations supporting the achievement of vitrification [48], and he and Meryman sought additional evidence. When the evidence came in, it showed that Luyet’s exemplary gelatin gels, formerly thought to have been vitrified, were actually crystalized [49, 50]. This result, the restriction of vitrification to only very tiny samples that could be cooled and warmed at very high rates [51], and presumably the availability of the alternative of colligative cryoprotection, all led to a waning of interest in vitrification. Today, in PubMed, there is no explicit citation of vitrification as a means of cryopreservation between 1950 and 1984. Notably, however, Bruggeller and Mayer established that a cooling rate of 105–106°C/s is sufficient for vitrification of dilute solutions in 1980 [28], and Dubochet and McDowall showed the absence of visible ice in quenched 1 micron thick aqueous films by cryo-electron microscopy in 1981 [52].
Cryoprotectant-Assisted Vitrification
In 1965, Farrant supercooled guinea pig uteri to dry ice temperature using 55% dimethyl sulfoxide (Me2SO) to prevent ice formation and recovered them in a viable condition [53]. He did not propose vitrification, but the next year, a series of seminal studies began to show that similarly high concentrations of cryoprotectants enable not only supercooling but actual vitrification, even at low cooling rates [54–58]. In 1968, Rapatz and Luyet cooled erythrocytes rapidly in ~5.3 M [2] glycerol and saw no ice either intracellularly or extracellularly and recovered the cells intact after rewarming [59], thus providing the first clear proof of successful vitrification. Rapatz, further inspired by Farrant [60], went on to apply similar methods to whole hearts. He ultimately showed that frog hearts could recover fully after supercooling to dry ice temperature with 10–11 M EG [61], but immersing them in liquid nitrogen resulted in fracturing upon rewarming [60]. Perhaps because of the setback previously experienced by Luyet, however, he always described the frog heart experiments as “freezing” experiments rather than as a way of approaching vitrification.
Boutron, unaware of Farrant and Rapatz’s work but inspired by the reports demonstrating vitrification of solutions, independently recognized, in 1978, that “in the extreme case of a solution which remains entirely amorphous even at very slow cooling or warming rates, all cells should be protected” [62]. This is the first statement of the modern approach to bulky system vitrification. He proceeded to obtain the first detailed data on the kinetics of ice formation as a function of solution composition and created the first mathematical models describing that information. He also defined the concepts of the critical cooling rate and the critical warming rate and developed the first measures of the critical warming rate. He recognized the danger of toxicity from high concentrations of cryoprotectants [63] and proposed to avoid this danger by finding solutions that could vitrify at low concentrations that might be less toxic [62]. Although he initially found that dilute solutions have impossibly high critical warming rates (1.7 × 104 and 7 × 107 °C/min for 45% w/w Me2SO and 50% w/w glycerol, respectively), he later discovered the remarkably strong glass-forming properties of 1,2-propanediol (PG) [64] and 2,3-butanediol (2,3-BD) [65] and ultimately showed that red cells can be vitrified and rewarmed in PG [66] and 1,3-butanediol (1,3-BD) [67] with survival.
In 1980, James independently conceived of complete vitrification and tested the idea with some success [68]. James and Farrant previously found that the worm, Schistosoma mansoni, could survive in small proportions after pretreatment with methanol, slow freezing to −30°C, and plunging into liquid nitrogen and proposed that they survived because the unfrozen 40% methanol–water solution remaining in the worms at −30°C would vitrify during the plunge to −196°C [69]. This inspired the following thought: “If successful cryopreservation of schistosomula depends on vitrification of the intracellular contents, it should be possible to achieve this more simply by using a high cryoprotectant concentration and rapid cooling. The initial slow cooling step could thus be omitted and the chance of the damaging effects of extra and intracellular ice formation eliminated” [68]. And in fact, worms treated with 40% methanol for 1 min and quenched survived about 10% of the time upon rewarming at 10,000°C/min. Lower concentrations and higher concentrations of methanol failed, presumably due to inadequate protection from ice damage at lower concentrations and from toxicity at higher concentrations. Success required a cooling rate similar to the cooling rate of > 1200°C/min required to vitrify 40% methanol, and the fastest warming rate possible. However, even higher survival (23%) was obtained when exposure to methanol was for only 10 s, implying that any methanol permeation into the worms may have been slight. But even if the worms were fully saturated with methanol after 10 s, their critical warming rate would have still been greatly in excess of 10,000°C/min (see later).
The work of Rapatz, Boutron, and James theoretically enabled a new and better form of vitrification, but their ideas were not widely pursued at the time, perhaps in part because they were obscure, awkward, and unconnected to systems that were of interest to large numbers of investigators. It would take a more practical departure from the ideas promulgated in the 1930s to alert the broader cryobiological community to the possibility of a new approach to cryopreservation.
Cryoprotectant-Assisted, Low Cooling Rate Vitrification
Unaware of Boutron’s and James’ work, but inspired by Farrant and Rapatz, I was searching for a way of preserving organs by extreme supercooling in 1979–1980 [11, 70] and suddenly realized that extending supercooling all the way to TG might enable whole organ vitrification [9, 27, 70–73]. This idea required the use of very concentrated solutions, which meant that cryoprotectant toxicity had to be confronted head on. Fortunately, I was able to formulate a number of effective means of mitigating toxicity (reviewed in [2, 27]) and, by 1983 [74], had removed toxicity as a clear barrier to success [24]. What was then missing was a proof of principle applied to a system of practical significance.
Meanwhile, in 1980–1983, Bill Rall’s incisive cryomicroscopic observations were showing that slowly frozen embryos survive as a result of intracellular vitrification [75–77]. This enabled Bill to immediately realize that the idea of complete vitrification [74] was not so different from partial vitrification, and should therefore work. We joined forces circa 1984 at the American Red Cross lab in Bethesda and were soon able to show that embryos could survive vitrification at both high and low cooling rates, provided warming was fast enough to prevent devitrification [18]. The results provided, finally, the first definitive evidence that vitrification is capable of reproducibly preserving complex mammalian systems with very high survival rates. Soon, the race was on to tailor vitrification methods for use on all manner of cells and tissues.
Simultaneously with the work of Rall and Fahy, Takahashi, Hirsh, and colleagues, working in the same Red Cross lab and using the same VS1 solution (except for the carrier), were investigating the feasibility of vitrifying human monocytes [32]. Their paper, published in 1986, reported that vitrification resulted in essentially no reduction in cell numbers or cell functionality unless devitrification was permitted to occur, and confirmed both the absence of ice upon cooling and the formation of ice upon devitrification using cryo-scanning electron microscopy and differential scanning calorimetry. In the course of these studies, they collected some of the only data available even today on the loci and sizes of ice crystals formed as a result of different extents of devitrification, the relationship between the extent of devitrification and cellular injury, the relationship between cellular injury and the size of intracellular ice crystals formed during devitrification, and the relationship between the warming rate and the extent of calorimetrically measured devitrification. Specifically, in VS1, the amount of devitrification was seen to decline logarithmically to almost zero as the warming rate increased from 2 to 80°C/min [29, 32].
Minimally Cryoprotectant-Assisted, Extreme Cooling Rate Vitrification and “One-Way” Vitrification
The same year Rall and Fahy demonstrated the general feasibility of vitrification, Peter Mazur and Peter Steponkus were selected by the National Science Foundation in the United States to develop a method for cryopreserving Drosophila melanogaster embryos [78]. They both eventually succeeded by using a vitrification approach employing 8.5 M ethylene glycol (EG) and cooling rates of ≥24,000°C/min to outrun chilling injury [13, 14]. Ultimately, Mazur reported that success was more dependent on the warming rate than the cooling rate, suggesting incomplete permeation of the embryos with EG [13], but it was still clear that Drosophila could survive only after vitrification and not after freezing and thawing [13] due to overwhelming chilling injury with the latter. This represented an important milestone in vitrification history, and focused attention on the possibility of vitrification at very high cooling rates, but nevertheless with a requirement for adequate pretreatment with cryoprotectant.
This approach was perhaps first extended to the problem of chilling injury in mammalian systems by Martino, Songsasen, and Leibo in 1996 [15]. Cooling bovine oocytes on copper microscope grids after treatment with either 5.5 M EG + 1 M sucrose or 4 M EG + 0.5 M sucrose was successful even though the latter solution, cooled in straws, did not vitrify. The authors speculated that even if extracellular ice were to form in the latter case, it might not matter. However, there is no reason to think ice formation would be restricted to the extracellular space in such a protocol. This raises the question as to whether some protocols succeed by, and should be referred to as, vitrification, whereas others are actually only rapid freezing protocols in which death is avoided by sufficiently rapid warming, and should be referred to as such.
The approach described by Martino et al. was followed by many protocols that emphasize high cooling and warming rates and minimize the cryoprotectant concentration (listed in [20]). It is important to recognize that, in at least some of these valuable methods, devitrification upon rewarming, whether visible or not [1], may be inevitable. These methods may be referred to as “one-way vitrification” because vitrification takes place on cooling but the amorphous state is not maintained on warming [1]. One-way vitrification lies between complete ice avoidance and rapid freezing with survival of IIF due to rapid warming. The need for extremely high warming rates in many rapid cooling protocols involving marginal concentrations of cryoprotectants (e.g., [19, 79]) presumably reflects the conditions required for survival of IIF arising either during cooling or during warming.
A few words are in order about recent efforts to vitrify sperm without the use of cryoprotectants. Although survival has been reported, survival alone does not establish vitrification, and direct evidence such as an absence of visible ice in cryo-scanning electron microscope images [32, 52, 59] has been lacking [1]. Although the cells may survive, a correct understanding of cryopreservation requires the ability to factually describe the fate of cells subjected to tested procedures.
Physical Principles of Vitrification
The Concentration and Temperature Dependence of the Stability of the Amorphous State
Vitrification is possible because both extreme temperature reduction and the presence of cryoprotectants inhibit ice crystal nucleation and growth. The general effects of these factors can be diagramed using a supplemented or “dynamic” phase diagram such as the one described in Figure 6.2 for the glycerol–water system. A true phase diagram describes equilibrium states, but dynamic events can be depicted on such a diagram by, for example, representing events that take place at a fixed cooling rate or a fixed warming rate. In Figure 6.2, all lines other than TM show the temperatures at which events are observed at a cooling or warming rate of 10°C/min.
Figure 6.2 Dynamic phase diagram for glycerol in water. Tm, Th, Td, and Tg depict the temperatures of melting, homogeneous nucleation, devitrification, and the glass transition, respectively. X designates a heterogeneous nucleation event. Cv, Cndv, and Cu are as defined in the text; Ce represents the eutectic concentration (although the eutectic is almost never seen in practice). PCG refers to the existence of a “partially crystallized glass.” For discussion, see text.
Both TM and TH decrease as the cryoprotectant concentration increases, and TG increases. As a result of this, at some concentration, CV, it becomes possible to cool from TM to TG and below without traditional homogeneous nucleation. At lower concentrations, in region I, cooling almost invariably leads to heterogeneous nucleation events (denoted by Xs), and TH can be reached only when heterogeneous nucleation is prevented (which usually requires sample emulsification into small droplets). However, apparent vitrification is possible at concentrations below CV, especially in region II, because in this region, the rates of both heterogeneous and homogeneous nucleation greatly diminish, and any nuclei formed from homogeneous nucleation remain too small to detect directly owing to an extreme suppression of ice crystal growth due to the very low temperatures at which the ice nuclei form.
This phenomenon is illustrated in Figure 6.3, which shows the temperature ranges permitting significant rates of ice nucleation and growth [25] in the organ vitrification solution (VS), M22 [80]. For solutions in regions II and III of Figure 6.2, the temperature ranges for appreciable nucleation and ice growth overlap minimally or not at all, so cooling results in nuclei too small to visualize or to cause biological injury, but when the solution is rewarmed, rapid ice growth becomes possible, and devitrification is observed. With more dilute solutions, the temperature ranges for nucleation and growth overlap, and outright ice formation is seen upon cooling unless ultrarapid cooling is applied.
Figure 6.3 Temperature dependence of the relative rates of ice nucleation and ice growth in the M22 vitrification solution. With permission from [11]
Although apparent vitrification can be achieved in region II, TH > TG and the resulting glass is therefore heavily nucleated and is thus referred to as a “doubly unstable” or “partially crystallized glass” [24]. This is manifested in the most dilute areas of region II by the appearance of the TD curve very close to TG, despite extremely low rates of ice growth at such temperatures. This can only be possible because of the existence of astronomical numbers of nucleation sites, each of which requires practically no ice growth to result in rapid conversion of all freezable water into ice near TD. As concentrations increase and nucleation density decreases, TD increases until, at some concentration (CNDV), devitrification is no longer observed. This point defines the onset of region IV, which is the region in which ice formation is possible essentially only via the growth of pre-existing ice in the solution (which, during slow freezing, occurs along the extension of the TM curve into region IV). At a sufficiently high concentration (CU), the solution becomes completely unfreezable, even in the presence of pre-existing ice, and the solution is virtually stable (region V).
The Cooling Rate Dependence of the Stability of the Amorphous State
As the cooling rate increases, TH decreases and TG increases, extending the amorphous state to lower concentrations. However, depicting this effect in a figure like Figure 6.2 is limited by a lack of adequate data. Therefore, other methods have been used to define the stability of the amorphous state as a function of concentration, cooling rate, and warming rate.
Boutron [37] first successfully described the kinetics of ice formation on cooling using a single equation that pertains to all solutions and all solution concentrations, each of which can be characterized by a single number k4 and knowledge of the maximum possible mass fraction of ice that can form at low cooling rates (qmax). This equation is particularly pertinent to events in region II of Figure 6.2, which is the region of most current interest in reproductive cryobiology. The Boutron equation is:

where x varies from 0 to 1 and is the ratio of ice mass crystallized on cooling to qmax, v is the cooling rate, and k4 is an empirical constant. When plotted, x decreases in sigmoid fashion as v increases, and the critical cooling rate, vcc, is the value of v that makes x close to zero. For example, if vcc is defined as the cooling rate that brings the total amount of ice formed to 0.2% of the mass of the aqueous sample, the Boutron equation simplifies to [39]:

and if one wishes to define vcc as the rate that brings x to 10–6, then [39]

In general, for any chosen value of x, the cooling rate required to achieve it is a linear function of k4.
Figure 6.4 shows the critical cooling rates of a variety of cryoprotectant solutions [81, 82] as a function of concentration, and an extrapolation of available data for glycerol and PG to concentrations approaching zero [83]. These rates will be affected by the presence of other solutes such as sugars (including those present in carrier solutions) and cytoplasmic solutes, and therefore represent upper limits. For example, the mass percent CV of solutions of EG and monosaccharides depends on the total mass of solute and not on the mass of EG alone [84]. Nevertheless, the rates in Figure 6.4 provide a valuable frame of reference for evaluating both claims of vitrification and cooling systems intended to achieve vitrification.
Extra-colligative Enhancement of the Stability of the Amorphous State
The discussion earlier describes the effects of cryoprotectants that do not display specific interactions with ice and affect ice nucleation and growth essentially indirectly, based largely on their concentrations and effects on solution viscosity and water content. There are, however, specialized molecules that are able to inhibit ice nucleation and crystal growth through specific molecular interactions with ice-like structures (“ice blockers”). Particularly in large volume, low cooling rate vitrification, a few resistant ice nucleation centers can require a significant increase in CV to achieve an ice-free state, so a search was made for agents capable of directly inhibiting such nucleation events by adsorbing to ice-like structures [85]. The first interesting agents identified were 1,3-cyclohexanediol [22], variants of polyvinylalcohol [86], and polyglycerol [87], which were able to slow ice growth and augment the thermal hysteresis effect of antifreeze proteins [22], lower CV by 3–5% w/w [86], or lower the temperature of spontaneous freezing at high temperatures and specifically block bacterial ice nucleating activity [87]. Since these early studies, two other candidate “ice blockers” have shown promise in vitrification experiments [88, 89], and other agents may have future applications for vitrification enhancement [1].
The Warming Rate Dependence of the Stability of the Amorphous State
A mathematical relationship between the critical cooling rate (vcc) and the critical warming rate (vcw) was recently proposed [2]. In particular,

where x = vcc. Available data are depicted in Figure 6.5 and show amazingly tight fits and surprising relationships between similarly-behaving cryoprotectants. Data for glycerol (G) are insufficient, but glycerol seems to behave like 2,3-BD at low vcc values. Although the curves plateau at high vcc, the critical warming rate remains extremely high compared to the critical cooling rate.
Figure 6.5 Relationship between the critical warming rate and the critical cooling rate for four families of cryoprotectants. Abbreviations as in Figure 6.4 and the text.
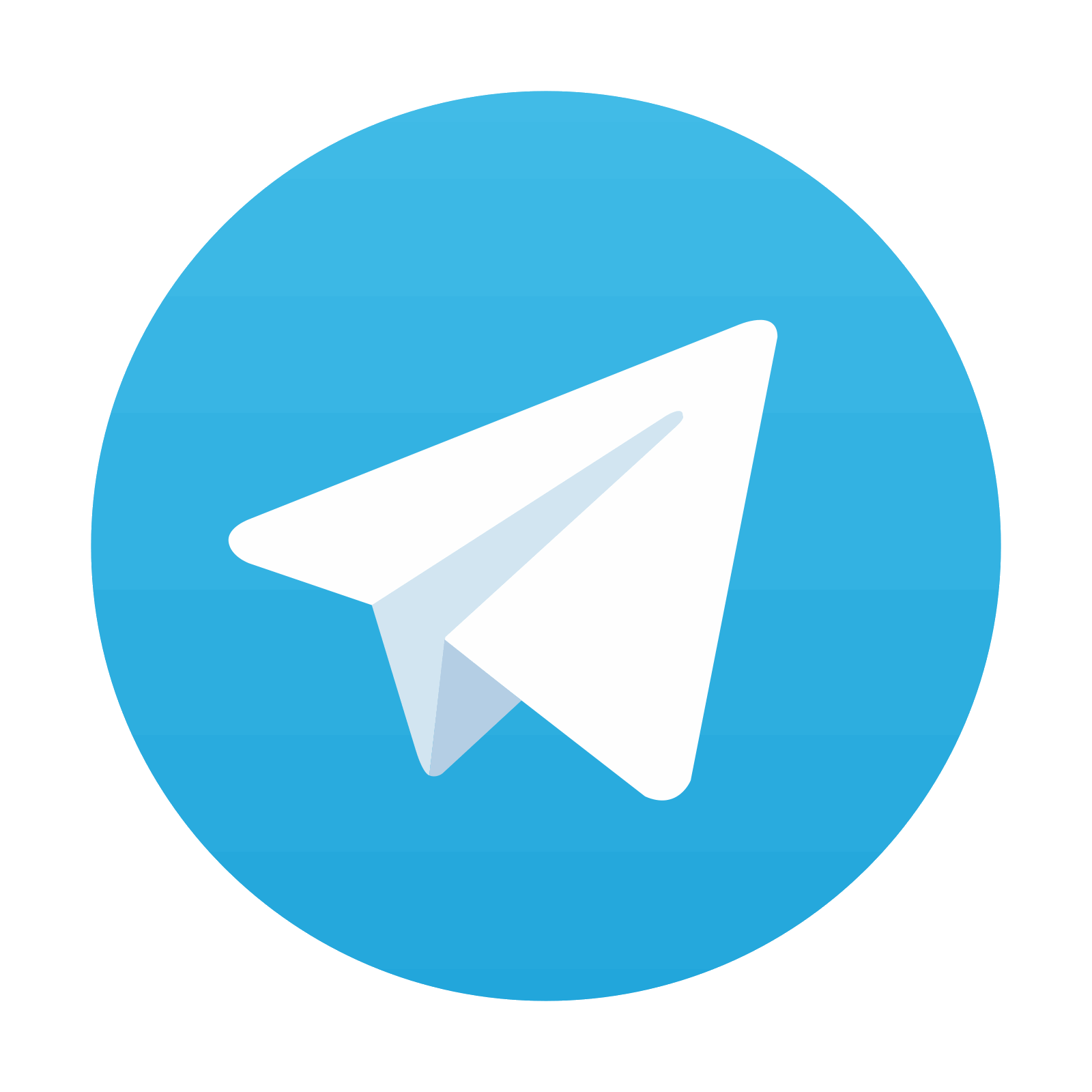
Stay updated, free articles. Join our Telegram channel
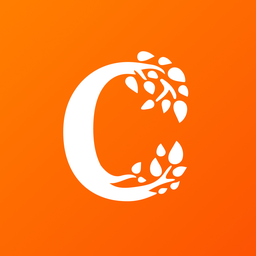
Full access? Get Clinical Tree
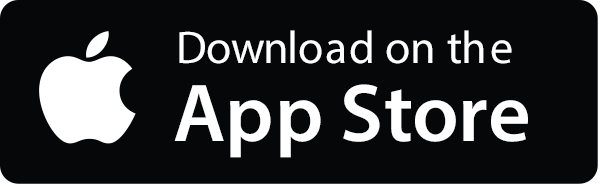
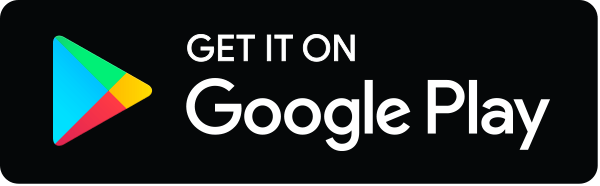