Abstract
In the first reported case of IVF and embryo development in the human, Rock and Menkin made the following description of the culture and development of a fertilized embryo: “The eggs were incubated in serum for 22.5 hours, being washed in salt solution before and after incubation, and then exposed to a washed sperm suspension in Locke’s solution for two hours at room temperature. They were again washed in Locke’s solution and cultured in fresh serum for 45 hours.
Introduction
In the first reported case of IVF and embryo development in the human, Rock and Menkin[1,2] made the following description of the culture and development of a fertilized embryo: “The eggs were incubated in serum for 22.5 hours, being washed in salt solution before and after incubation, and then exposed to a washed sperm suspension in Locke’s solution1 for two hours at room temperature. They were again washed in Locke’s solution and cultured in fresh serum for 45 hours. When examined at the end of the incubation period, one egg was found to be in the two-cell stage; two blastomeres of fairly uniform size and appearance, and containing granular cytoplasm, were enclosed within a zona pellucida along the border of which were numerous spermatozoa. At least one of them was clearly seen within the zona.” Thirty years later, following considerable effort and research, the first IVF birth was described by Steptoe and Edwards in 1978. Many landmarks occurred in the three decades intervening these two reports. In the ensuing 40 years since the birth of Louise Brown, some 70 years after the initial studies of Menkin and Rock, dramatic advances in knowledge and technologies have been made in the IVF laboratory, facilitating live birth rates after transfer to surpass the 50% mark in some patient groups and oocyte donors. The crucial laboratory based advances that have helped these rates to be achieved include ICSI, embryo cryopreservation through vitrification, blastocyst culture, and PGT. What will the next 10–20 years hold and how will the IVF laboratory of the future look?
The IVF laboratory, the cornerstone of successful IVF, will play an increasingly important role in the coming years in improving live birth rates, but also ensuring that the children born from IVF procedures are healthy and not affected by the procedures themselves[3]. The current laboratory workflow comprises numerous steps (Figure 5.1). This chapter elaborates on the current IVF laboratory workflow and how these steps could be performed in an IVF cycle of the future.
Andrology
Sperm Analysis, Preparation, and Selection
One of the first interfaces of the laboratory with IVF patients is the analysis of the semen sample. Remote analysis of a semen sample has already been described in a number of scenarios. This may be as simple as a sample being sent in a solution to a central laboratory from a patient’s home[4] or a patient being able to perform an analysis at home by creating a smartphone video, which will provide information on sperm quality[5]. As will be a consistent theme in the future laboratory, all aspects of the patient’s procedure will be accessible through secure access to a patient’s individual internet portal (see Chapter 9). This initial semen analysis could provide the basis for the male’s future treatment with their partner.
The home-based analysis will provide analysis of sperm concentration, motility, motility patterns, and morphology. A sometimes-forgotten component of semen analysis that may be performed remotely is assessment of oxidative stress. A number of tools are now available to provide further information about semen quality and male infertility. One system, the Male Infertility Oxidative System, is the latest advancement in laboratory assessment of oxidative stress and can establish an oxidative reproductive potential value that is capable of differentiating normal from abnormal semen and fertile from infertile men[6]. Further information on sperm quality, plausibly needed as a marker of child health, will involve molecular analysis of sperm characteristics that should define whether couples will proceed directly to IVF/ICSI or have an equal chance of achieving pregnancy using insemination. Specific methylation and micro RNA profiles of sperm characteristics could be examined to ascertain whether the semen profile of the male will negatively impact success rates. Numerous groups have already shown that the unique nature of the sperm epigenome and the patterns found in mature sperm that appear to reflect perturbations in spermatogenesis may ultimately have an effect on pregnancy outcomes[7–9]. It is believed these traits will provide predictive insight that can be exploited as a diagnostic tool. Indeed, there is emerging data suggesting that the predictive power of DNA methylation and RNA signatures in sperm likely exceeds that which can be found with traditional assessments of male infertility[10–12]. The future andrology laboratory will therefore be equipped with molecular diagnostic capabilities to assess the sperm epigenome as a diagnostic tool in the context of male infertility. Much of the testing will be done in house and analysis likely provided remotely via secure connections.
Sperm preparation will also be performed in a manner that will be aimed at identifying populations of or single sperm that have limited or no risk of passing on paternal traits that could affect fertilization, embryo development, pregnancy, and the health of the offspring. Human epidemiological studies have suggested a relationship between advanced paternal age at conception and adverse neurodevelopmental outcomes in offspring, including an increased risk for psychiatric conditions such as autism and schizophrenia[11]. In a recent study, over 40 million documented live births were examined by the Centers for Disease Control and Prevention and the National Center for Health Statistics database between 2007 and 2016 in the United States[13]. The study investigated the impact of advanced paternal age on maternal and perinatal outcomes and found a higher paternal age was associated with an increased risk of premature birth, low birth weight, and low Apgar score. Although as of the time of writing clear evidence is not available that sperm selection technologies provide dramatic improvement in improving pregnancy rates, a common theme in such studies has been reduction in miscarriage rates.
How will sperm be prepared and selected in the future? The most promising methodology will likely involve selection via a microfluidic platform for IVF/ICSI cases[14]. These platforms have already entered into a testing phase in many IVF laboratories[15]. For example, in the early 2000s, Takayama and Smith used two parallel laminar flow channels where nonmotile spermatozoa and debris would flow along their initial streamlines and exit one outlet, whereas motile spermatozoa had an opportunity to swim into a parallel stream and exit a separate outlet. They found that both motility (98%) and morphology (22%) improved significantly[16].
Relying solely on the motility characteristics and size to sort sperm by microfluidics will most likely be surpassed by further innovations to these systems. Already, we have seen the addition of chemoattractants to further help separate sperm in a microfluidics platform[17,18]. Addition of cumulus cells in a microfluidic chamber, to act as the chemoattractant, helped increase the percentage of motile sperms from 58.5% to 82.6%[18]. A further addition will definitely be the integration of optics into the selection process[19]. One of the most interesting applications for integrating microfluidics and optics may be the use of Raman spectroscopy, which has already been shown to distinguish sperm with improved nuclear integrity[20].
The IVF Laboratory Processes
It is envisaged that several aspects of clinical embryology over the coming decades, including ICSI, embryo culture, and cryopreservation, have the potential to become automated. Such advances may be achieved through microrobotics and microfluidic systems, which are already being adapted into many processes of IVF (Figure 5.2).
Figure 5.2 Integration of microfluidic systems for (A) sperm selection, (B) egg collection, (C) insemination/ICSI, (D) embryo culture, and (E) cryopreservation. All stages are monitored by noninvasive microscopy involving time-lapse, image recognition, fluorescence-lifetime imaging microscopy (FLIM), and spectroscopy, while diagnostic ports are able to collect DNA, protein, and metabolites for analysis. Computer-controlled channels will allow input and output of fluids to allow gamete/embryo movement and to individualize culture conditions related to developmental stage. Channels will also allow control of cryopreservation to maximize vitrification and warming. All gamete and embryo data will be integrated with individual patient data to allow artificial intelligence (AI) to construct a reproductive health assessment of each embryo.
Egg Collection and Processing
The first step in an IVF procedure is to collect oocytes. There have been no automated and continuous processing systems that can reduce the dependence on well-trained embryologists to obtain ICSI-ready oocytes from patients. Recently, however, using mouse models, a microfluidic device to denude oocytes from the surrounding cumulus-corona cell mass was reported[21]. Oocytes that were denuded by the device showed comparable fertilization and developmental competence compared with mechanical pipetting. The device allows access to denuded oocytes that would be ready for either ICSI or vitrification.
ICSI and Routine Insemination
The advent of ICSI[22] revolutionized the treatment of the infertile male. Its adaptation in the IVF laboratory has surpassed its traditionally intended role and it is now applied to nearly 70% of cases worldwide[23]. In many clinics it is used in 100% of cases. The automation of this skill has been attempted by various groups in recent years[24]. In particular, the group of Sun in Toronto has used a robotic system to track sperm and inject them with variable success[25]. None of these systems have been clinically adopted as yet. However, another approach could be via the use of microfluidics.
Although the use of ICSI has been adopted on a more regular basis, the use of microfluidics may allow more improved access of sperm to oocytes in smaller chambers, similar to that seen in the oviduct or fallopian tube. In fact, microfluidics may better mimic oocyte sperm interactions in drastically reduced volumes (mimicking those present in the female tract), allowing for fertilization rates to be achieved with minimal numbers of surrounding spermatozoa. Han and colleagues[26] developed a microwell array used to capture and hold individual oocytes during the flow-through process of oocyte and sperm loading, medium substitution, and debris cleaning. Different microwell depths were compared by computational modeling and flow washing experiments for their effectiveness in oocyte trapping and debris removal. Fertilization was achieved in the microfluidic devices with similar fertilization rates to controls. The use of shorter exposure and low sperm numbers for insemination has long been argued to improve embryo quality[27] and the use of these systems may translate to improvements in not only process but outcomes.
Embryo Culture
Our ability to culture preimplantation embryos has improved dramatically over the last 40 years. Incubators, culture media, and culture environment have significantly improved and can now facilitate development of the fertilized oocyte to the blastocyst stage in all laboratories[28,29,30]. Although many aspects of embryo culture have improved, the actual hardware we culture embryos in has not changed. Standard culture conditions still call for an embryo to be grown in a petri dish using relatively large media volumes, when compared to embryo size, under oil. The major change in embryo culture in the past 10 years has been in the utilization of time-lapse systems, which has allowed clinics to assess embryos in a continuous temperature and gas phase without environmental perturbations. A number of studies have now shown that the time-lapse systems surpass the older standard incubators[31,32,33] indicating that one benefit may definitely be a nondisturbed environment[34].
One of the unperceived advantages that coincides with time-lapse incubation is the use of specifically designed culture wells that are able to better create microenvironments around the embryo. These wells are characterized by lower incubation volumes per embryo, and by their columnar design in which embryos reside in the base of a small column of medium. These changes to dish design alone have been shown to improve embryo development, compared to that seen in a conventional petri dish in drops of medium under oil[35].
The concept of a nondisturbed environment for culture lends itself well to microfluidic culture systems. Various microfluidic platforms have already been tested in human IVF programs[36]. Some of the original studies reported by Heo and colleagues[37] cultured mouse embryos in a microfluidic system and found that blastocyst development was significantly enhanced in development to the hatching stage and in the average number of cells per blastocyst. Importantly, dynamic microfluidic culture (in which embryos moved) significantly improved embryo implantation and OPRs over static culture to levels approaching that of in utero derived preimplantation embryos[36]. Unfortunately, although many systems have been developed that allow microfluidic embryo culture to be performed, their adaptation has yet to be realized. However, this could change in the future as the microfluidic platforms also allow two major adjunct improvements to embryo culture. Firstly, they allow the manipulation of the media so that it can better suit the dynamic nature of preimplantation embryo development[38]. The adoption of single-step culture media, although perceived to be equivalent to sequential, does not cater to the embryos’ real metabolic needs. Microfluidics will allow these metabolic needs to be synchronized more closely to embryo stages. For example, we envisage a future where embryos are monitored individually and, as they progress in development, they are exposed to changing gradients of nutrients to maximize the physiology of each stage[39]. This could be performed for multiple factors including carbohydrates[40], amino acids[28,41,42], vitamins, growth factors, cytokines[43], and macromolecules. More importantly, interactive culture systems could be developed whereby embryo culture may finally be able to mimic that of an in vivo environment. In a recent study by Ferraz and colleagues[44], bovine embryos were cultured in a microfluidic chamber in addition to oviductal feeder cells. The authors concluded that cultured embryos were able to show in vivo characteristics as culture using “oviduct-on-a-chip”. This culture system yielded 50% of zygotes with no discernible difference in gene expression pattern when compared to in vivo zygotes. They postulated that their results highlight the importance of a more in vivo like environment when studying pathways related to normal fertilization and zygote formation in vitro and suggested that future studies should focus on the relevance of this improved environment for further (epi)genetic reprogramming events in developing embryos.
A second benefit of microfluidic platforms is the ability to perform in line diagnostics of embryos as they are growing. This concept was proven by Urbanski and colleagues[45], who showed that a microfluidic device could be employed in which media samples are loaded or integrated into microfluidic culture systems for in-line, real-time metabolic evaluation. As a proof of principle, metabolic activities of single murine embryos were evaluated using this device. Interestingly, they also adapted the use of a standard epifluorescent microscope and a charge-coupled device camera to their system, which opens up further possibilities of incorporating other diagnostic technologies covered later in this chapter.
Embryo Cryopreservation
Since the first report of a frozen IVF pregnancy in 1983 by Trounson and Mohr[46], embryo cryopreservation has become a major part of any IVF cycle. Although embryo freezing was able to provide limited successes, the advent of vitrification has revolutionized freezing of eggs and embryos to the extent that frozen embryo transfers are now surpassing fresh transfers as an option for ET[47,48]. In the future it is plausible that the current benefits seen in the transfer of vitrified embryos will translate to all cycles being completed by a frozen ET. In addition, change in clinical practice may provide a better luteal support and improve live birth outcomes[49].
The challenges to improving freezing protocols are related to both time and outcome efficiency. Automated vitrification systems are already under development, which are aimed at improving laboratory efficiency[50,51]. A further improvement will be the ability to provide greater control over the cryopreservation process. For example, the group of Sun[52] has described a digital microfluidic device that automates sample preparation for mammalian embryo vitrification. The automated operation allowed gametes or embryos to pass through a cryoprotectant concentration gradient, which is distinct from current practice where they are passed through several steps of solutions. This in itself may improve outcomes. Further refinements may also involve the ability to visually monitor individual eggs or embryos during the cryopreservation process and adjust the addition of cryoprotectants to each egg or embryo, which may be of greatest significance in a population of oocytes from older patients. In the future, therefore, we may provide “personalized egg or embryo freezing” through a combination of optical and microfluidic techniques.
Egg and Embryo Analysis
The development of precise methods for assessing oocyte and embryo quality has long remained a critical goal in ART. Forty years after the first IVF procedures were performed, morphological assessment remains the primary method of evaluation, despite its well-known limitations[53]. Morphological features per se, however, have no clear connection to the underlying biochemical factors that are essential for viability, such as metabolic function and genetic integrity[54]. However, using time lapse to quantitate the timing of key events, such as initiation of cavitation to full blastocoel expansion, could well be linked to metabolic activity over a defined period[53]. Current technology for embryo assessment is largely based on biopsy of the trophectoderm followed by genetic analysis. This, however, has raised a number of concerns not only because the procedure is invasive and requires removal of cells from the embryo, but also because it is only representative of part of the embryo. We consider that in future these tests will be superseded by noninvasive testing, which will give a more holistic evaluation of the entire embryo and will provide information about genetic, metabolic, and transcriptional activity, thereby not only reflecting the ploidy and viability of the embryo but providing important information regarding the health of the embryo itself[3].
Metabolic
The metabolic state of an embryo is not only predictive of viability (defined as the ability to implant) but is also reflective of the actual health of the embryo (as defined by the development of the resultant fetus). To date, assessment of metabolic function has relied heavily on specialized miniaturized procedures, available to only a few laboratories worldwide. Noninvasive embryo assessment of the embryo’s metabolic state can now be performed indirectly through a number of novel imaging platforms, which can obtain information about the metabolic functions of the embryo. Raman spectroscopy, Fourier transform infrared spectroscopy, or FLIM and hyperspectral analysis can all be used to provide additional spectroscopic data on embryos, and they thus have the potential to produce superior clinical tools for embryo assessment[55,56]. One methodology that has recently provided interesting data on mouse eggs and embryos is FLIM[57]. Interestingly, experiments on mouse oocytes have shown that FLIM parameters exhibit strong differentiation between old versus young oocytes. FLIM could potentially be used as a noninvasive tool to assess mitochondrial function in oocytes and can also discriminate between oocytes that have had their mitochondria genetically manipulated. Other types of microscopic analysis are also being examined in the realm of embryology. Hyperspectral analysis investigates overall autofluorescent signatures and their cellular distribution. Like FLIM, this technique has also been shown in other cell systems to provide insights into cellular processes, including embryos. Recently, this type of analysis was shown for the first time to extract noninvasively, biologically relevant, and quantitative information from cells and tissues. It was able to identify and document autofluorescence by multispectral imaging whereby a spectrum is taken at each pixel in the image, generating about a million such spectra from cellular areas with varying molecular composition[57]. Individual cells are segmented out and their images processed to generate multiple, mathematically defined cellular features. The endogenous cell fluorophores examined included nicotinamide adenine dinucleotide, nicotinamide adenine dinucleotide phosphate, flavin adenine dinucleotide, flavin mononucleotide, N-retinylidene-N-retinylethanolamine, cytochrome C, and proteins including abundant species like collagen and elastin. The ability of these novel optical methods may be integrated in egg and embryo assessment in the future laboratory. It is plausible that such systems will be combined with microfluidic platforms to provide real-time embryo analysis.
Genetic Noninvasive/Semi-Invasive PGT
At the time of writing, the most reliable embryo analysis technology is PGT-A using trophectodermal biopsies. This technology consistently provides greater than 50% live birth rates when a euploid embryo is found for transfer (see Chapter 2). Unfortunately, the technique requires significant laboratory resources, including the actual trophectoderm biopsy itself and the addition of cryopreservation of all blastocysts prior to analysis. Recently, however, the advent of noninvasive PGT-A may circumvent much of the need for biopsying embryos. Promising noninvasive methods to genetically analyze the embryo include blastocoelic fluid[58] and/or media assessment[59,] and the use of advanced microscopy techniques[60,61]. In several years’ time, the need to biopsy an embryo will most likely be unnecessary.
AI
Through the introduction of time-lapse microscopy, we are now in the unique position of collecting more data than a clinical embryology laboratory can utilize. With embryos being imaged every 10 minutes, during a 4- to 5-day culture period, over 700 pictures (each with several focal planes) are amassed, therefore taking the total number of images available for consideration into the thousands (and to think we used to look at embryos once each day, giving a total of 5 or so images on which to make our decisions!). With the ability to annotate embryo development, and the application of algorithms to model the significance of discrete events, we can now utilize the potential of time-lapse to a greater extent than first imagined[54,62,63]. However, even the most sophisticated algorithms to date use only a small percent of the available information. AI is a means to maximize the potential of time-lapse microscopy in clinical IVF[64]. Rather than taking a sample of images into consideration, AI can utilize every image at every focal plane, in order to learn what time-dependent features are associated with successful transfer outcome, such as fetal heart rate[65]. Furthermore, AI will be of value in integrating the growing amount of clinical data collected per embryo, allowing patient parameters to also be combined into decision-making with the images acquired throughout development.
A final benefit of AI in combination with microfluidics will be added safety for tracking patients’ gametes and embryos, allowing all the data to be assimilated into electronic medical records, which would include witnessing systems. This will allow patients’ gametes and embryos to be tracked more efficiently with the ultimate aim of eliminating any errors in the laboratory[66].
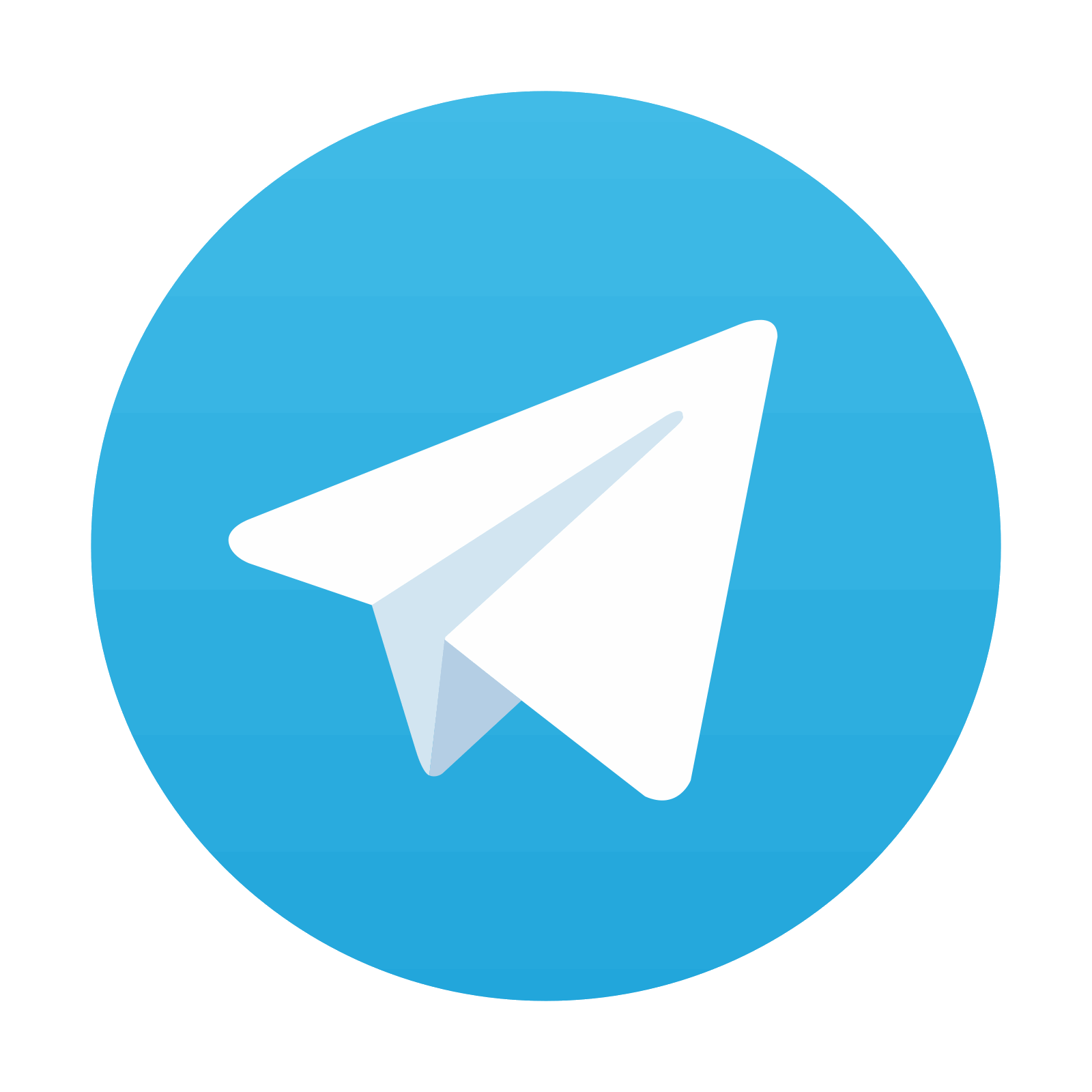
Stay updated, free articles. Join our Telegram channel
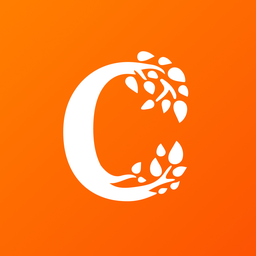
Full access? Get Clinical Tree
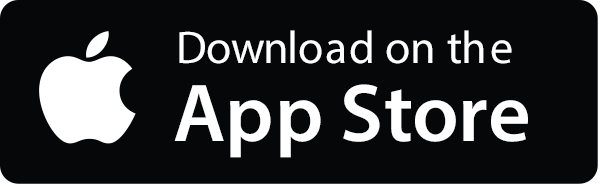
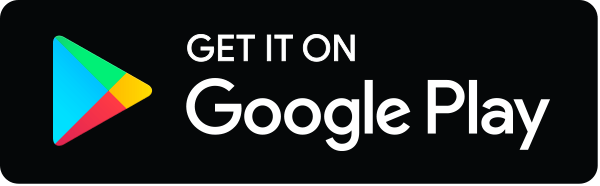