Abstract
Apoptosis, a physiological process for the controlled deletion of cells, is critical for the regulation of cell numbers, the management of morphogenesis during embryonic development, and the orchestration of many cellular processes in the adult. Spermatogenesis, the production of functional spermatozoa from spermatogonial stem cells, is no exception. It appears that a functional apoptotic pathway is necessary for normal spermatogenesis to develop, and without it infertility ensues. Apoptosis also plays a crucial role in the maintenance of the testis and its response to external toxicants, as well as in the programmed senescence of terminally differentiated spermatozoa. This chapter focuses specifically on how apoptosis affects sperm quality and function, and the implications of this process for both embryonic development and the health and well-being of the offspring.
Introduction: Apoptosis and DNA Damage in Human Spermatozoa
Apoptosis, a physiological process for the controlled deletion of cells, is critical for the regulation of cell numbers, the management of morphogenesis during embryonic development, and the orchestration of many cellular processes in the adult. Spermatogenesis, the production of functional spermatozoa from spermatogonial stem cells, is no exception. It appears that a functional apoptotic pathway is necessary for normal spermatogenesis to develop, and without it infertility ensues. Apoptosis also plays a crucial role in the maintenance of the testis and its response to external toxicants, as well as in the programmed senescence of terminally differentiated spermatozoa. This chapter focuses specifically on how apoptosis affects sperm quality and function, and the implications of this process for both embryonic development and the health and well-being of the offspring.
A great deal of data has accumulated in recent years suggesting that human spermatozoa can exhibit some of the hallmarks of apoptosis including activation of caspases 1, 3, 8, and 9 [1, 2], annexin-V binding [3, 4], mitochondrial generation of reactive oxygen species (ROS) [5], and DNA fragmentation [6–8]. The latter is potentially extremely important because DNA damage in the male germ line has been associated with a wide variety of adverse biological and clinical outcomes. Thus DNA damage in human spermatozoa has been correlated with poor fertilization and impaired embryonic development to the blastocyst stage [9, 10] as well as with the incidence of subsequent miscarriage [11–13]. Furthermore, even if such pregnancies do carry to term, the presence of DNA damage in the spermatozoa at the moment of conception has been linked with developmental abnormalities in the offspring leading to a wide range of different pathologies, including childhood cancer [12].
DNA Damage in Spermatozoa, Assisted Reproductive Technology and Embryonic Development
This apparent association between DNA damage in spermatozoa and the health and well-being of any progeny is particularly significant in the context of assisted reproductive technology (ART), which has come to dominate the therapeutic landscape for infertile couples. Thus, the developed nations of the world are currently seeing an exponential increase in the use of ART to treat human infertility to such an extent that 1 in 80 children born in the United States, 1 in 50 born in Sweden, 1 in 40 born in Australia, and 1 in 24 born in Denmark are the product of this form of treatment. In 2003, more than 100,000 in vitro fertilization (IVF) cycles were reported from 399 clinics in the United States, resulting in the birth of more than 48,000 babies [14, 15]. Worldwide, this figure has now exceeded 200,000 births per annum and is continuing to rise. This massive uptake of ART may herald a developing public health crisis for at least two reasons. First, human infertility is a complex multifactorial condition that is strongly impacted by genetic factors that ART will ensure are passed onto the progeny. As a consequence, it is a biological certainty that the more we use assisted conception to treat one generation, the more we shall need it in the next. Second, in at least half of the patients referred for ART, there is a problem with the male partner. Since male subfertility is frequently associated with high levels of DNA damage in spermatozoa, the use of ART invariably means that DNA damaged cells will be used to achieve conceptions in vitro that could never have occurred in vivo. This raises genuine concerns over the safety of IVF procedures should the DNA damage brought into the zygote by the fertilizing spermatozoon subsequently lead to disordered embryonic growth and development [15, 16].
We already know that the incidence of birth defects following assisted conception is double than seen in the naturally conceived population [17], and there is good evidence for an increase in imprinting disorders, notably the Beckwith–Wiedemann and Angelman’s syndromes, in such children [18, 19]. Infants produced by ART are also significantly more likely to be admitted to a neonatal intensive care unit, to be hospitalized and to stay in hospital longer than their naturally conceived counterparts [20]. Large studies of Scandinavian populations using record linkage have also shown an increase in the hospitalization of ART offspring in infancy and early childhood compared with spontaneously conceived children [21–23]. Such increases in morbidity cannot be completely explained by multiple births because singletons are similarly affected [23]. Furthermore, recent independent investigations have shown an eightfold increase in the incidence of undescended testicles in boys conceived by intracytoplasmic sperm injection (ICSI) [24], while another study has demonstrated abnormal retinal vascularization in such children [25]. ICSI has also been associated with a slight, but significant, increase in autism rates [26]. Notwithstanding the concerns raised in these publications, it must also be stressed that other authors have been more reassuring about the normality of ICSI children, particularly in terms of their motor skills and cognitive development [27–28]. Such a mixed picture for the safety of ART arises from the literature for a number of reasons:
1. The incidence of definable events such as overt birth defects, hospitalizations or significant pathologies such as cancer is low.
2. The study populations are generally small.
3. The number of confounding variables influencing the health and well-being of children is great.
4. The duration of follow-up is short.
5. The instruments used for assessing defects in the embryo (incidence of miscarriage, birth defects, overt changes in the health and behavior of the offspring) are insensitive.
If we assume that the genetic/epigenetic damage associated with ART is randomly distributed throughout the genome then the chances that a specific gene will be directly affected is low, given that 95% of the genome is noncoding. It is even less likely that if a mutation does occur in a gene, it will be dominant and cause a detectable phenotypic or behavioral change in the F1 generation. Clinically, the incidence of even the more common spontaneous dominant mutations, such as achondroplasia, approximates to 1 in every 10,000–30,000 births in the population at large [29]. Thus, if phenotypic change is the criterion, then most ART studies are simply not adequately powered to determine whether this form of therapy has a significant effect on the normality of the offspring. In this situation, the “absence of evidence” cannot be taken as the “evidence of absence” of problems associated with ART, and we should proceed on the assumption that this form of therapy carries significant risks that have to be quantified and addressed. The notion that DNA damage in human spermatozoa constitutes part of this risk is supported by clinical evidence generated around two major paradigms: aging and smoking.
Age, DNA Damage, and Disease in the Progeny
As men age, they do not stop producing spermatozoa. However, the quality of the gametes they do produce deteriorates, leading to a loss of fertility [30] and increased levels of DNA damage in the spermatozoa [31]. The latter has been consistently observed to correlate with age across a number of studies, using a variety of different methods to detect the DNA strand breaks. Thus Singh et al. employed a Comet assay to demonstrate a significant correlation between age and DNA damage in human spermatozoa [31]. In the same vein, Schmid et al. found older men to have increased alkali-labile sites or single-strand DNA breaks in their spermatozoa using the same assay [32]. Others have employed the sperm chromatin structure assay (SCSA) to demonstrate a similar relationship between DNA damage and male age [33]. Furthermore, both human and rodent data have revealed age-related increases in the number of spermatozoa with chromosomal breaks and fragments [34]. A detailed analysis of chromosome 1 also found that the frequency of sperm carrying breaks, segmental duplications, and deletions was significantly higher among older men compared with their younger counterparts [35]. In particular, the frequency of spermatozoa carrying breaks within the 1q12 fragile-site region nearly doubled in older men. In contrast to female gametes, there was no effect of age on the frequency of sperm with numerical chromosomal abnormalities [35].
This abundant evidence for an effect of paternal age on DNA damage in the germ line is important because a father’s age appears to be a major determinant of the health and well-being of his offspring. Indeed, for many decades we have been aware that paternal age has a dramatic influence on the incidence of spontaneous dominant genetic mutations such as achondroplasia, Apert’s syndrome (acrocephalosyndactyly), and multiple endocrine neoplasia. For example, an analysis of 154 consecutive cases of dominant genetic mutations to determine the parent of origin revealed that in every single case the mutation could be traced back to the male germ line, never the female. Moreover, the appearance of these mutations was found to be an exponential function of the age of the father. Such mutations appear to be created by replication error in the spermatogonial stem cell population, followed by clonal expansion of mutant germ cells as they enter a “selfish” pathway of proliferation [36, 37]. Of course, the genetic damage occurring in human spermatozoa as a function of paternal age is not only associated with dominant genetic mutations. Age is also associated with an increase in the incidence of complex polygenic neurological conditions in the offspring including epilepsy, spontaneous schizophrenia, bipolar disease, and autism [12, 38–41], as well as an increased rate of death in the F1 generation associated with congenital malformations, injury, and poisoning [42].
It is clear from the foregoing that paternal age is associated with high rates of DNA damage in spermatozoa and that such age-dependent genetic damage to the male gamete is, in turn, associated with a wide range of clinical pathologies in the offspring, from dominant genetic mutations to complex neurological disorders. This age-dependent DNA damage is thought to be caused by a variety of mechanisms including replication error, chronic exposure to environmental toxicants, and oxidative stress originating from a combination of diminished antioxidant protection and elevated levels of ROS generation by the spermatozoa [43, 44]. Oxidative stress also appears to be at the heart of another factor known to increase DNA damage in the male germ line, that is, smoking.
Smoking, DNA Damage, and Disease in the Progeny
Heavy paternal smoking is thought to place the body under systemic oxidative attack causing a significant depletion of antioxidant vitamins such as vitamins C and E [45–47]. One of the consequences of the resultant stress is thought to be DNA fragmentation and formation of the oxidized base adduct 8-hydroxy-2’-deoxyguanosine (8OHdG) in the spermatozoa [46]. In addition, benzo[a]pyrenes derived from cigarette smoke can form adducts with sperm DNA, once metabolically activated by the cytochrome P450 system [48]. The clinical significance of these sperm DNA adducts can be found in the positive correlations that have been repeatedly observed between paternal smoking and serious morbidity in the offspring, including childhood cancer [49–53].
Is DNA Damage Related to Apoptosis in the Germ Line?
In summary, the previous clinical data provide powerful circumstantial evidence that DNA fragmentation in human spermatozoa is correlated with adverse clinical outcomes ranging from miscarriage to cancer. These conclusions are also supported by animal data indicating that the experimental induction of oxidative DNA damage in spermatozoa with cigarette smoke, alcohol, or the experimental deletion of antioxidant enzymes, such as glutathione peroxidase 5, can subsequently lead to disruptions of embryonic growth resulting in high rates of abortion and the appearance of birth defects [54–56]. Given that these associations between sperm DNA damage and abnormalities of embryonic development clearly exist, it is now critical that we gain an understanding of how this DNA damage is generated in human spermatozoa and, in the context of this review, the role of apoptosis in the prosecution of this process.
Nature of the DNA Damage
In order to understand the origins of DNA damage in the male germ line, we first have to understand its precise nature. A small number of studies have actually examined the damaged DNA from human spermatozoa in the search for clues as to its origins. These studies have revealed that the major base adduct present in human spermatozoa is 8OHdG, a marker of oxidative stress. The levels of 8OHdG expression in human spermatozoa are consistently found to be elevated in the spermatozoa of infertile men [57, 58]. Moreover, the presence of this oxidized base adduct has been found to exhibit an extremely strong correlation with both DNA damage as measured by the TUNEL assay and chromatin protamination as assessed by chromomycin (CMA3) staining [57]. Indeed, the correlation between TUNEL and 8OHdG formation is so strong that we have been forced to conclude that a majority of DNA damage in the male germ line is oxidatively induced (Figure 5.1 [57]).
Figure 5.1 The powerful correlation observed by De Iuliis et al. [57] between DNA fragmentation in human spermatozoa and oxidative DNA base damage as indicated by expression of 8-hydroxy-2’-deoxyguanosine (8OHdG)
Besides 8OHdG, biochemical analyses of DNA from infertility patients have also revealed the presence of two ethenonucleosides: 1,N6-ethenoadenosine and 1,N2-ethenoguanosine.These compounds probably arise from a reaction with 4-hydroxy-2-nonenal, the main aldehyde released during lipid peroxidation [59]. These findings are again consistent with oxidative stress being a major factor in the etiology of DNA damage in the male germ line.
In another study, uncharacterized bulky DNA adducts were found to be significantly more common in the spermatozoa of male factor infertility patients than in a cohort of healthy donors [60]. Furthermore, a significant negative correlation was observed between these bulky DNA adducts and sperm concentration and sperm motility, among patients with impaired fertility [60]. In a further study, polycyclic aromatic hydrocarbon–DNA adducts were found to be more prevalent in infertile versus fertile men [61]. While the origin of some of the DNA adducts detected in human spermatozoa are clear, as in the case of smoking [48], we clearly still have a lot to learn about the causes of DNA damage in the germ line. The one thing that does appear to be certain is that a significant proportion of the spontaneous DNA damage seen in male infertility patients is oxidative in nature.
The link between DNA oxidation (8OHdG formation) and DNA strand breakage could be interpreted in one of two ways. First, the link may be causative. That is, the formation of oxidative base adducts disrupts DNA integrity by labilizing the glycosyl bond that attaches the base to the ribose unit with the resultant generation of an abasic site. Abasic sites have a strong destabilizing effect on the DNA backbone which can subsequently result in strand breaks. Alternatively, the relationship may be indirect. Oxidative base damage and DNA fragmentation may simply be independent witnesses to the same fundamental underlying process – the ability of spermatozoa to undergo apoptosis. Under these circumstances the DNA strand breakage could be linked to endonucleases activated during an apoptotic cascade that was triggered by oxidative stress. Such considerations have encouraged us to consider the process of apoptosis in the male germ line as these cells differentiate from spermatogonial stem cells to fully formed spermatozoa.
Ability of the Germ Line to Undergo Apoptosis
The ability of germ cells to undergo apoptosis is expressed very early in life when the testes are differentiating and adjustments have to be made to achieve the optimal ratio of germ cells to Sertoli cells. During this developmental process, excess premeiotic spermatogonia are removed by an early wave of apoptosis that accompanies the first round of spermatogenesis in the testis. Functional deletion of the pro-apoptotic protein, Bax, or overexpression of anti-apoptotic factors such as BclxL or Bcl2, generates a male infertility phenotype associated with a perturbed germ cell to Sertoli cell ratio [62, 63]. This phenotype shows disordered seminiferous tubules filled with spermatogonia, but no mature haploid sperm, while other tubules lack any germ cells whatsoever. Later in life, p53 and Fas are involved in the removal of germ cells that are damaged as a result of exposure to environmental toxicants or chemotherapeutic agents [64]. A role for apoptosis in the etiology of spontaneous male infertility has also been suggested by virtue of the excessively high numbers of apoptotic germ cells observed in the testes of some infertile males [65]. It has also been suggested that the DNA damage that features so prominently in human spermatozoa is the result of an abortive apoptotic process that was initiated during spermatogenesis but failed to run to completion because the extensive remodeling of germ cells to produce spermatozoa removes the intracellular machinery needed to effect cell death [66].
Significance of Spermiogenesis in the Etiology of DNA Damage
Spermiogenesis, the process by which round spermatids differentiate into spermatozoa, is a key event in the etiology of DNA damage in the male germ line. As indicated earlier, it is possible that some of the DNA breaks seen in spermatozoa are the result of an abortive apoptotic process initiated during spermiogenesis in response to some disruptive event. Equally, DNA fragmentation in spermatozoa may also be the result of unresolved strand breaks created during the normal process of spermiogenesis in order to relieve the torsional stresses involved in packaging a very large amount of DNA into a very small sperm head. Normally, these “physiological” strand breaks are resolved by a complex process involving H2Ax expression, formation of poly(ADP-ribose) (PAR) by nuclear poly(ADP-ribose) polymerases (PARP) and topoisomerase [67]. However, if spermiogenesis should be disrupted for some reason then the restoration of these cleavage sites might be impaired and the spermatozoa, lacking any capacity for DNA repair in their own right, will be released from the germinal epithelium still carrying the unresolved strand breaks.
Experimentally, if physiological strand break repair during spermiogenesis is impaired then spermatozoa are generated that exhibit high levels of DNA fragmentation. For example, it is known that the chromatin remodeling steps associated with spermiogenesis trigger PAR formation, as an early event in DNA repair. Knockout mice deficient in enzymes involved in PAR metabolism (PARP1 and/or PARG [poly(ADP-ribose) glycohydrolase, 110-kDa isoform]) display DNA strand breaks associated with varying degrees of subfertility [67]. Similarly the “transition” proteins that move into the sperm nucleus during spermiogenesis, between the removal of histones and the entry of protamines, are thought to play a key role in maintaining DNA integrity. If these proteins are functionally deleted then spermatozoa are generated with poor fertilizing potential, poor chromatin compaction, and high levels of DNA fragmentation [68]. Such studies clearly indicate that the functional disruption of chromatin repair mechanisms operative during spermiogenesis can result in the genesis of spermatozoa carrying high levels of DNA fragmentation. However, even though disruption of DNA repair mechanisms during spermiogenesis can result in DNA-damaged spermatozoa, this does not necessarily mean that such mechanisms are actually operative in a clinical context.
Using the fluorescent probe CMA3 to monitor the efficiency of sperm protamination, many independent laboratories have generated data on the excellent correlations observed between DNA fragmentation on the one hand and poor chromatin remodeling during spermiogenesis on the other (Figure 5.2a–c). This proposed link between defective spermiogenesis and DNA damage is further supported by the fact that several independent studies have recorded correlations between DNA damage in human spermatozoa and elements of the conventional semen profile (specifically sperm count and morphology) that, in turn, reflect the efficiency of the spermatogenic process.
Figure 5.2 The close relationship between the efficiency of chromatin remodeling, as monitored by chromomycin (CMA3) fluorescence and DNA damage in spermatozoa: (a) correlation between CMA3 and 8-hydroxy-2’-deoxyguanosine (8OHdG); (b) correlation between CMA3 and DNA fragmentation as measured by the TUNEL assay; (c) image of the TUNEL signals generated by human spermatozoa possessing damaged DNA (arrowed)
Not only is the disruption of spermiogenesis correlated with DNA damage; it is specifically correlated with oxidative DNA damage as reflected by 8OHdG formation (Figure 5.2). We postulate that this relationship exists because the poorly remodeled chromatin detected by CMA3 is particularly vulnerable to oxidative attack by ROS originating from a number of potential sources including infiltrating leukocytes, depleted antioxidant systems, and excessive free radical generation by the spermatozoa’s own mitochondria or alternative sources such as lipoxygenase [69]. Experimental conditions associated with the induction of high levels of oxidative DNA damage, such as exposure to radio frequency electromagnetic radiation (RFEMR) [9] or the triggering of apoptosis through the suppression of PI3 kinase with wortmannin [R. J. Aitken, unpublished observations], invariably involve the release of considerable amounts of ROS from the sperm mitochondria, although the contribution of alternative sources is currently unknown.
Importance of Chromatin Remodeling and Oxidative Stress
In light of the earlier considerations, DNA damage in human spermatozoa appears to have its origins in the testes and is associated with oxidative stress. We might interpret these data in two different ways:
1. Impaired chromatin compaction and oxidative DNA damage are independent events. It is possible that a variety of different factors are primarily responsible for impairing the quality of spermiogenesis (e.g., endocrine disruption, environmental toxicants, exposure to electromagnetic radiation or genetic mutations). The result of this disrupted spermiogenetic process is the production of spermatozoa with poorly remodeled chromatin that, because of the lack of DNA compaction, are particularly vulnerable to oxidative stress and the induction of 8OHdG formation. Under these circumstances, the oxidative stress could come from the variety of sources mentioned earlier, including impaired antioxidant defenses or the enhanced generation of free radicals by either the spermatozoa themselves or leukocytes in the immediate vicinity of these cells.
2. Impaired chromatin compaction and oxidative DNA damage have a common origin in oxidative stress. We have already cited evidence that DNA damage in spermatozoa is largely oxidatively induced. Whether spermiogenesis can also be adversely affected by oxidative stress is still a matter of debate. In male toxicology models involving, for example, the administration of streptozotocin [70], bacterial lipopolysaccharide [71], deltamethrin [72], methyl-parathion [73], aroclor 1254 [74], cyclophosphamide [75], or formaldehyde [76], oxidative stress is associated with the disruption of spermatogenesis via mechanisms that can be reversed by the administration of antioxidants such as lipoic acid [77], quercetin [70], Satureja khuzestanica essential oil [75], and melatonin [76]. In some of these experiments, the administration of an antioxidant both improved testicular function and reduced DNA damage in spermatozoa [75], suggesting that oxidative stress can underpin both the disruption of spermatogenesis and the induction of DNA damage in spermatozoa. In addition, the induction of oxidative stress with methyl parathion has been shown to specifically affect chromatin remodeling during spermiogenesis and induce DNA damage in spermatozoa [78]. Such results encourage speculation that oxidative stress is a major determinant of the efficacy of spermiogenesis which, when it becomes disrupted, results in the generation of spermatozoa that are vulnerable to oxidative stress, 8OHdG formation and, ultimately, DNA fragmentation ([79–80]). A powerful demonstration of the validity of this hypothesis has been provided by Zubkova and Robaire [81]. These authors induced systemic oxidative stress through the administration of the glutathione-depleting drug l-buthionine-[S,R]-sulphoximine (BSO). In response to the oxidative stress so generated, the rats exhibited evidence of both impaired spermiogenesis, as evidenced by an increase in CMA3 staining of the sperm chromatin, and increased DNA fragmentation.
Thus, oxidative stress can impair spermiogenesis and this, in turn, precipitates DNA fragmentation via a number of potential mechanisms (Figure 5.3):
1. Physiological DNA strand breaks introduced to facilitate DNA folding during spermiogenesis are left unresolved and persist in the mature gamete.
2. The defective spermatozoa generated as a result of disrupted spermiogenesis possess poorly protaminated chromatin that is vulnerable to free radical attack generating 8OHdG adducts, abasic sites and, ultimately, DNA fragmentation.
3. The defective spermatozoa, generated as a result of disrupted spermiogenesis, respond to oxidative stress by entering a default apoptotic pathway that results in endonuclease-mediated DNA cleavage.
Importance of Apoptosis in Spermatozoa
There is no doubt that spermatozoa can exhibit many of the features of apoptosis and there is good evidence that oxidative stress can trigger apoptosis in these cells. Thus, exposure of human spermatozoa to hydrogen peroxide (H2O2) can readily trigger an apoptotic cascade characterized by the activation of caspase 3 and the appearance of annexin-V binding positivity [82]. We have also demonstrated that the activation of an apoptotic cascade following H2O2 exposure results in the induction of mitochondrial free radical generation [83]. The activation of mitochondrial ROS generation, in turn, induces the formation of 8OHdG adducts in human sperm chromatin. We have observed this cascade of cause-and-effect involving oxidative stress, the activation of mitochondrial ROS generation and the induction of oxidized base adduct formation following: (1) the direct addition of oxidant (H2O2) to spermatozoa; (2) the indirect creation of oxidative stress with RFEMR [84]; and (3) the activation of apoptosis using the PI3 kinase inhibitor, wortmannin [83]. We have also pursued this apoptotic cascade to determine whether the activation of caspases, mitochondrial ROS generation, and phosphatidylserine (PS) externalization is followed by the activation of endonucleases that then move into the sperm nucleus to induce DNA fragmentation.
Surprisingly, this analysis revealed that apoptosis in spermatozoa differs in one important respect from this process in somatic cells, in that even though endonucleases may be released from the mitochondria (endonuclease G, apoptosis inducing factor) or activated in the cytosol (caspase-activated DNase) during the apoptotic cascade, the physical architecture of these cells prevents these nucleases from translocating to the sperm nucleus for two reasons:
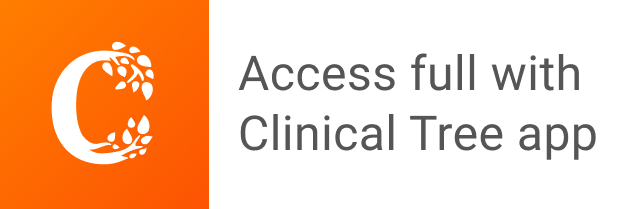