Abstract
The sperm epigenome is unique and of profound clinical importance based on its role in influencing embryogenesis. Potentially, the sperm epigenome can also be of importance to the clinician and toxicologist in assessing the effects of environmental exposures and lifestyle factors on spermatogenesis and sperm function, since the sperm epigenome contains historical markers of spermatogenesis, as well as programmatic factors of early embryonic development. Various industrial and agricultural chemicals are implicated in affecting male fertility, and recent studies have begun to evaluate the effects of these chemicals on the sperm epigenome. Additionally, lifestyle factors, such as obesity and advanced paternal age at the time of conception, are also being shown to affect the sperm epigenome. This chapter evaluates such factors and their effects on the sperm epigenome, highlighting the possible effects on both the father and potential progeny.
4.1 Introduction
Epigenetics is the field of study of heritable factors, other than DNA base pair coding, that regulate gene expression. It is important to emphasize the heritable component of epigenetics, both in terms of the definition and in terms of the scope of epigenetic effects, since by definition epigenetic alterations not only affect the health of the primary individual but also may have transgenerational effects on future progeny [1]. Hence, in a world in which individuals are increasingly exposed to industrial chemicals, environmental pollutants, and lifestyle changes such as increased obesity and advanced ages of paternity, epigenetic perturbations potentially induced by these types of environmental exposures are of prime importance in both individual and societal health concerns [2–5].
The male gamete is unique in its specialized structure and functions. The mature sperm cell is terminally differentiated to provide a mechanism by which the paternal genome can be transported through the female reproductive tract, undergo biochemical and morphological changes that facilitate fertilization of the oocyte, then undergo profound chromatin remodelling events during syngamy. One aspect of the sperm cell’s uniqueness is its epigenome, both a historical record of the pathway of spermatogenesis, as well as a blueprint for early embryogenesis events [6–9]. Not only are key spermatogenesis genes marked in specific patterns that reflect spermatogenesis fidelity, but key developmental genes are bivalently marked to “poise” the genes for timely expression during embryogenesis. This pattern appears to be conserved in nature, and has been shown to be associated with poor embryo development and infertility.
In this chapter, we briefly review the basic aspects of the sperm epigenome, including DNA methylation, histone modifications, and non-coding RNAs (ncRNAs). We then highlight the known effects of some lifestyle and environmental influences on the sperm epigenome, highlighting the potential ramifications to the reproductive and general health of the male and his future progeny.
4.2 Overview of the Epigenome
The basic epigenetic factors that exist in spermatozoa are DNA methylation, histone and chromatin modifications, and ncRNAs (Figure 4.1). These epigenetic markers demonstrate extreme plasticity throughout sperm development as a consequence of cellular reprogramming and have also been shown to be modifiable by environmental conditions, as will be discussed in Section 4.3. There are two main epigenetic reprogramming events during mammalian development: the first one occurs in primordial germ cells (PGC) in the early stages of spermatogenesis, and the second reprogramming event occurs following fertilization, at the onset of embryogenesis [10,11]. These reprogramming periods are potential windows of susceptibility for the development of aberrant epigenetic programming. Aberrations in the sperm epigenome can lead to defects throughout spermatogenesis, thereby reducing fertilization capabilities and pregnancy rates. Evidence also suggests that alterations in the sperm epigenome may lead to defects or failures in embryonic development, miscarriage, or inheritance of paternal disease phenotypes in offspring [6,12].
4.2.1 Sperm DNA Methylation
DNA methylation exists throughout all stages of mammalian development. Methylation is a critical modification that provides regulation of tissue-specific gene expression patterns. In the male gamete, methylation is responsible for genomic imprinting, a mechanism for generating parent-of-origin specific gene expression in early embryos [13,14]. In mammals, DNA methylation consists of the covalent addition of a methyl group at position 5 of the cytosine ring, resulting in the formation of 5-methylcytosine (5meC). This epigenetic modification occurs mainly in cytosine-phosphate-guanine (CpG) dinucleotides and is initiated and maintained by DNA methyltransferases (DNMTs). CpG islands are usually found at gene promoters, and can prevent gene expression when hypermethylated by preventing access of transcriptional machinery to the DNA strand.
Sperm DNA is relatively hypermethylated in comparison to the hypomethylated patterns seen in oocytes, but is actively demethylated following fertilization prior to methylation patterns being reset in the developing embryo [15]. Several studies have investigated associations between changes in sperm DNA methylation patterns and male fertility. Changes in sperm methylation markers may imply a number of changes in spermatogenesis, leading to a decrease in sperm quality [16]. Moreover, DNA methylation patterns are involved in control of the functional capacity of germ cells and these alterations may affect fertilization and pregnancy rates [17]. It has also been shown that sperm methylation patterns can be predictive of male fertility and embryo quality during in vitro fertilization treatment, since methylation levels at specific regions were reduced significantly in the infertile men analyzed [18].
4.2.2 Sperm Histone Modifications
In order to protect sperm DNA from damage and deliver paternal nuclear content to the oocyte, sperm chromatin is organized in a very compact structure in which sperm DNA is wrapped around protamines as opposed to histones. During the late stages of spermatogenesis, sperm cells undergo dramatic chromatin structural rearrangements and approximately 85–90% of histones are replaced by protamines. In mammals, the protamination process occurs in two steps, first with a substitution of canonical histones by transition proteins, and then subsequent replacement by protamines 1 and 2 (P1 and P2) [19]. Protamines are capable of facilitating the very tight, toroid structure that sperm DNA forms by modulating interactions between themselves and the DNA sequence. In order to bind to DNA, protamines must be phosphorylated, and then, for DNA condensation, protamines are dephosphorylated, resulting in a chromatin structure 6 to 20 times more condensed than the classical somatic nucleosome [20]. In normal fertile men, the ratio of P1:P2 is tightly regulated and is approximately 1:1 [21]. Aberrant P1:P2 ratio is associated with abnormal semen parameters, DNA fragmentation, and reduced fertilization capabilities [22–24].
After the histone-to-protamine transition, the remaining 5–10% of histones were first considered remnants of an inefficient replacement process, but several studies have shown that histone retention does not occur randomly. Histones appear to be retained at specific genomic loci, such as developmental gene promoters, genes encoding microRNAs, and imprinted loci, which are regions related to fertilization and important to the developing embryo [25,26]. The modifications of histone proteins, via modification of lysine and serine residues on tail regions, promote activation or inactivation of gene transcription, a critical epigenetic regulator [17]. For example, modifications like acetylation of H3 and H4, ubiquitination of H2B, or trimethylation of H3K4, H3K36, and H3K79, create a more open chromatin structure, which can lead to gene activation [27]. On the other hand, deacetylation of H3 and H4, ubiquitination of H2A, and methylation of H3K9 and H3K27 can generate transcriptional inhibition [15].
4.2.3 Sperm Non-coding RNAs
It was thought that RNA transcripts present in sperm were just RNAs stored after meiosis or produced in the early stages of spermatogenesis. However, in recent years, different types of RNAs present in human spermatozoa have been described, as well as their activity in sperm development, fertilization, embryogenesis, and epigenetic inheritance [28–30]. Recent studies provide evidence that the spermatozoa RNA signature undergoes reprogramming during epididymal transit [31,32]. Sperm isolated from the caput epididymis show reduced abundance of RNA species that are found in the testes and cauda epididymis. These results indicate that sperm undergo either a random or programmed post-testicular loss of RNA species, and subsequent gain of ncRNAs during epididymal transit mediated by epididymosomes [31] (Figure 4.2).
Figure 4.2 The sources of the sperm RNA pool. This figure demonstrates that species of RNA molecules originate in two diverse regions of the testis. First, mRNA fragments and small RNAs are added in the seminiferous tubule throughout spermatogenesis. During transport though the epididymis, removal of some RNAs appears to occur in the caput epididymis, with subsequent replacement as the sperm traverse the epididymis. This replacement occurs via epididymosomes, exosomes containing miRNAs, tRFs, and various proteins.
Spermatozoal ncRNAs represent important epigenetic factors that may act as controllers for gene expression at the transcriptional and post-transcriptional level [29]. The most abundant RNAs found in sperm are the piwi-interacting RNAs (piRNAs) [28]. These are single stranded RNAs (24–30 nucleotides) that are associated with PIWI proteins and are responsible for transposon silencing. piRNAs predominantly contribute to spermatogenesis and embryogenesis in specific stages [33], and altered piRNA profiles could result in reduced male fertility, and may be related to testicular cancer [34,35]. miRNAs are also found in sperm and are transcripts that are 19–25 nucleotides long and promote gene silencing by binding to target mRNAs, leading to either mRNA degradation or translational repression. Sperm miRNAs have been associated with apoptosis, mitochondrial membrane integrity, failures in spermatogenesis and embryogenesis, and idiopathic male infertility [36–40]. Further studies are essential to confirm these hypotheses and to provide a better understanding of the role of these ncRNAs in embryo development and paternal inheritance.
4.3 Endocrine Disruptors, Male Fertility, and the Sperm Epigenome
A global trend in the reduction of male fertility has prompted research into potential environmental conditions that may play a role in male infertility [4,41]. Exposure to toxins in the form of pesticides, air pollution, and pharmaceutics has become increasingly common. Endocrine disruptors are a class of chemicals that exist in the environment due to many industrial and agricultural uses. Included in this class are agricultural and industrial chemicals such as DDT, dioxin, and plastics (i.e. bisphenol A, bis(2-ethylhexyl)phthalate, dibutyl phthalate). These chemicals are termed “endocrine disruptors” because of their ability to either mimic natural hormones in the body, inhibit the action of hormones, or alter normal functioning of the endocrine system [42].
4.3.1 Epidemiologic Evidence
Evidence from epidemiologic studies has suggested an association between males exposed to endocrine disruptors, either in utero or as adults, and reduced fertility [43,49]. Studies in mouse models have shown that embryonic exposure to certain endocrine disruptors such as vinclozolin result in male mice displaying increases in sperm cell apoptosis as well as other reproductive-related phenotypes [50–57]. Additionally, these mice produce sperm with alterations in DNA methylation patterns at promotors of multiple genes [52,55–57]. These observations prompt questions as to how exposure to these chemicals is capable of affecting fertility, and what consequences exist for offspring of exposed individuals.
Multiple reproductive phenotypes have been observed in men in association with endocrine and disruptor exposure. These include reduced sperm concentration, poor sperm motility and morphology, infertility, increased risk of testicular cancer, increased incidence of cryptorchidism, and others. Bibbo and colleagues reported reduced semen parameters such as sperm concentration in the sons of women treated with diethylstilbestrol, a synthetic estrogen previously prescribed to women to prevent miscarriage [43]. Polychlorinated biphenyl (PCB) metabolites and heavy PCB exposure in adult men has also been associated with poor semen parameters [44,45]. PCBs were once widely used in many applications as heat transfer fluids and coolants. Together, this evidence suggests that endocrine disruptor exposure, both in utero and in adulthood, has the potential to affect male fertility.
In addition to reduced fertility, individuals exposed to endocrine disruptors display increased risk of other reproductive-related disorders. Plastics workers can be exposed to multiple endocrine disruptors, such as bisphenol A (BPA) and bis(2-ethylhexyl)phthalate (DEHP). Increased incidence of testicular cancer has been observed in plastics workers, suggesting a role for endocrine disruptors as a risk factor for testicular cancer in adult men [46]. Additionally, based on a high prevalence of testicular cancer in specific populations and concurrent high dietary exposure to endocrine disruptor ochratoxin A in these populations, this chemical has been hypothesized as an additional risk factor for testicular cancer [47]. Increased incidence of cryptorchidism has also been observed in the sons of women working with pesticides, many of which have previously contained endocrine disruptors [48]. This suggests again that both in utero and adult exposure to certain endocrine disruptors may increase risk for reproductive-related disorders in men.
4.3.2 Evidence in Model Systems
In addition to the epidemiologic evidence that exists regarding endocrine disruptors and male reproductive effects, studies in model systems have been conducted. These studies have allowed the characterization of both phenotypic and epigenetic modifications occurring in male offspring of endocrine disruptor exposed pregnant females. Interestingly, research in mice has shown reduced fertility in male progeny transgenerationally following embryonic exposure to endocrine disruptors [58].
Multiple studies in mice have shown a host of phenotypic changes such as reduction in sperm cell counts and cell viability, increases in male infertility, testis disease, prostate disease, obesity, and pubertal abnormalities in the male offspring of pregnant females treated with endocrine disruptors such as vinclozolin, methoxychlor, and plastics compounds [50–54,56–58]. Interestingly, many of these phenotypes are observed in males from F1, F2, F3, and, in some cases, F4 litters. These studies provide evidence that the detrimental effects of endocrine disruptor exposure are not limited to exposed animals, but that these effects may be inherited transgenerationally by an epigenetic mechanism. It is important to note that for this inheritance to be considered “transgenerational,” the effects must be seen at least through the F3 generation. This is due to potential exposure of the in utero (F1) animal’s germ cells to the endocrine disruptor treatment during development. These potentially exposed germ cells give rise to F2 animals, therefore, if effects are only shown through the F2 generation, it is possible that effects are due to germ cell exposure and not inheritance from the F1 animal [50].
An interesting study involving the transgenerational inheritance of endocrine-disruptor mediated effects recorded the incidence of adult-onset prostate abnormalities in male offspring of vinclozolin exposed females. Vinclozolin exposure during the period of F1 embryonic gonadal sex determination resulted in the development of adult-onset prostate disease in males from the F1 to F4 generation. These prostate abnormalities included prostatitis, hyperplasia of the ventral prostate, and epithelial cell atrophy. Analysis of the prostate and ventral prostate epithelial cell transcriptomes revealed significantly altered expression of 954 and 259 genes, respectively, in the F3 generation. Included in these genes are MSP and FADD, which have both previously been associated with prostate disease and cancer. Additionally, other affected genes are involved in pathways such as calcium and WNT signaling [52]. These data suggest that endocrine disruptor exposure, during specific periods of embryonic development, is capable of inducing epigenetic transgenerational abnormalities in the prostate transcriptome and male germ line.
In addition to transcriptome changes and infertility and disease phenotypes observed in offspring of exposed animals, alterations to the sperm epigenome of F1 and F3 animals have been recorded. Specifically, regions of differential DNA methylation have been observed at promotors of multiple genes [50,55,57,59,60]. Interestingly, some of the genes associated with the differentially methylated regions are involved in a functional connection network involved in cell signaling pathways [50,57]. Due to the ability of some regions of paternal DNA methylation to partially escape reprogramming events in the early embryo, it is possible that altered germ cell DNA methylation is at least partially responsible for the incidences of epigenetic inheritance observed. In fact, some of the differentially methylated regions identified overlap imprinted genes known to escape epigenetic reprogramming [59,60]. Additionally, it has been hypothesized that changes in the germ line epigenome of early generations may induce genetic or transcriptome changes, such as observed copy number variants and gene expression changes, respectively, in later generations [52,55].
With the knowledge that endocrine disruptor exposure is associated with alterations to the sperm epigenome, Manikkam and colleagues aimed to determine whether DNA methylation alterations were consistent between exposures to different types of endocrine disruptors. They exposed pregnant females to pesticides, dioxins, and plastics, separately, in order to characterize differences in F3 sperm DNA methylation. Differentially methylated regions were observed for dioxin, plastics, and pesticide groups. Interestingly, there was very limited overlap between the differentially methylated regions recorded for each group. These data suggest that individually each endocrine disruptor may have a unique effect on the sperm epigenome in F3 animals [56,58]. This observation is especially interesting considering the wide range of reproductive phenotypes that have been observed in the male offspring of exposed females, ranging from infertility to pubertal abnormalities to testicular or prostate disease.
Additional alterations to the epigenome have been recorded following embryonic exposure to endocrine disruptors in the mouse. Vinclozolin exposure to pregnant females resulted in an increase of germ cell apoptosis, as previously described, as well as a reduction in PGC numbers, resulting in reduced fertility. These abnormalities were present in F1 to F3 generation males. Analysis of PCG miRNA populations in all generations revealed altered expression of multiple miRNAs, including miR-23b and miR-21, which induced alterations in the Lin28/let-7/Blimp1 pathway responsible for regulating PCG differentiation. These data suggest an epigenetic mechanism of transgenerational inheritance centered around the deregulation of miRNAs involved in cell differentiation [54].
The transgenerational phenotypic effects of embryonic endocrine disruption have been well characterized, and it is clear that an epigenetic mode of inheritance must be at least partially responsible for these observations. The recorded epigenome alterations, however, are often inconsistent between studies and between various endocrine disruptors. It is possible that different endocrine disruptors have unique effects on the epigenome and resultant unique phenotypes associated with their exposure, leading to the mosaic of reproductive phenotypes that have been observed. It is also likely that a combination of different epigenetic factors are responsible for the phenotypes observed [56,58]. Therefore, additional research on exposure is needed in other areas of the sperm epigenome, specifically in spermatozoal RNAs which have recently been strongly implicated as a mechanism for epigenetic inheritance. A more complete picture of sperm epigenome alterations as a result of embryonic endocrine disruptor exposure will also help to determine what cell types, tissues, and functions are acted upon following exposure.
4.3.3 Targets of Endocrine Disruptors in the Male Reproductive Tract
Many potential target sites exist for endocrine disruptors to act on male reproduction. Importantly, hormones produced in the testis are essential regulators of spermatogenesis. Therefore, endocrine disruptor action within the testis is a potential explanation for reduced fertility in exposed males. Leydig cells are found adjacent to seminiferous tubules within the connective tissue between tubules and are the primary endocrine cells within the testis. Production of testosterone by Leydig cells is under hormonal control by the pituitary. As the primary endocrine cells within the testis, these cells are potential targets for endocrine disruptor activity that may affect reproduction [42,61,62].
Sertoli cells form the inner lining of the seminiferous tubules and have important functions for sperm development. These cells function as endocrine cells, with the release of hormones inhibin and follicle stimulating hormone (FSH), which are important for the regulation of sperm development. Sertoli cells have additional functions, which may be perturbed by endocrine disruptor activity. These functions include the destruction of defective sperm cells, the provision of fluids important to sperm transport, and the provision of nutrients necessary for sperm development. Additionally, Sertoli cells are the basis for the blood–testis barrier, which is responsible for maintaining separation of the specialized tubule lumen environment from plasma components as well as preventing passage of agents, which may be toxic to sperm cells, into the tubule lumen. Disturbance of any of these regulatory or protective mechanisms, individually or in concert, by endocrine disruptors could be responsible for some of the epigenetic and phenotypic effects observed in exposed males [42,62].
As described, there are multiple cell types and structures within the testis which are potential targets for endocrine disruptor activity. In addition to these testicular structures, it is possible that endocrine disruptors have a direct effect on germ cells during development. During spermatogenesis, immature spermatogonia undergo mitotic divisions to become primary spermatocytes. These spermatocytes undergo meiosis to generate haploid spermatids, which condense and elongate and are eventually released as spermatozoa into the seminiferous tubule lumen. These cells then travel to the epididymis where final maturation and storage occurs prior to ejaculation. There are multiple stages during development, such as mitotic arrest, where developing germ cells are especially susceptible to toxic agents and damage. Additionally, the sperm epigenome undergoes significant remodeling during development and maturation, including changes to chromatin structure, development of DNA methylation patterns, and multiple waves of spermatozoal RNA payload remodeling [6,19,25,31,42]. These periods of susceptibility provide potential windows for endocrine disruptors to act directly on germ cells, leading to the phenotypic alterations observed as well as epigenetic alterations, which are likely involved in inheritance of these traits.
4.4 Age, Environmental Factors, Lifestyle Factors, and the Sperm Epigenome
4.4.1 Age and the Sperm Epigenome
Aging is associated with changes in dynamic biological, physiological, environmental, psychological, behavioral, and social processes that result in a decline in function of the senses and an increase of the susceptibility to diseases [63]. It is well known that aging is related to a decline in the male reproductive system, sperm quality, and fertility [64]. Also, recent evidences demonstrate a role for paternal aging on offspring disease susceptibility [65]. Notwithstanding these robust epidemiological evidences, in most cases the mechanisms that drive these processes are unclear, although it is thought that the sperm epigenome is possibly the key [66].
In that sense, one study analyzed sperm DNA methylation from fertile sperm donors in which semen was collected in the 1990s and again in 2008, and identified 139 regions that are significantly hypomethylated and 8 significantly hypermethylated regions associated with aging. Analyzing these regions, the authors found that the affected regions were associated with genes linked with schizophrenia and bipolar disorder, establishing the first non-causative relationship between paternal aging and the incidence of neuropsychiatric and other disorders in the offspring [67]. Moreover, Atsem and colleagues in a cohort study with 162 sperm DNA donors described two genes (FOXK1 and KCNA7 genes) with negative correlation between sperm methylation and paternal age. Also, the study suggested that the levels of methylation of the FOXK1 gene were transmitted into the next generation [68].
Both studies suggest that age-related DNA methylation changes in sperm may contribute to increased disease risk in offspring because changes can be transmitted to the next generation, and found that changes accumulate gradually with aging, not in a sudden precipice at a given age. These changes are particularly relevant with the known societal changes in regards to advancing paternal age generally.
4.4.2 Environmental Factors, Lifestyle Factors, and Sperm Epigenome
Male reproductive health is declining year by year, raising serious concerns and implications about human fertility [69]. There are multiple possible causes of this decline; however, the most plausible seems to be related to environmental and lifestyle factors such as psychological stress, air quality and pollution, unhealthy diets, overweight or obesity, and smoking and alcohol consumption.
4.4.2.1 Psychological Stress
Physiological stress can be defined as an organism’s response (e.g. biochemical, physiological, or/and behavioral changes) to a stressor such as an environmental condition. Some clinical studies reveal an inverse relationship between psychological stress and some quality semen parameters [70,74]. However, the relationship between male infertility and psychological stress is still controversial.
The hypothalamic–pituitary–gonadal (HPG) axis is one of the major systems that respond to stress disrupting endocrine homeostasis, mainly increasing or decreasing testosterone levels in the testes, affecting Sertoli cells that leads arrest of the spermatogenesis process and alters sperm quality [75]. However, it seems that this physiological stress also can affect the sperm epigenetic signatures, not only through DNA methylation changes, but also at the miRNA level.
There is only one longitudinal non-clinical study conducted to date that examined the association of psychological stress with DNA methylation in gametes [76]. In that study, combined physical, emotional, and sexual abuse in childhood was characterized in 34 men. The authors identified 12 DNA methylation regions differentially methylated by childhood abuse that contains genes associated with neuronal function (MAPT, CLU), fat cell regulation (PRDM16), and immune function (SDK1). These results confirmed that childhood abuse is associated with sperm DNA methylation changes and suggested that this may have implications for offspring development. However, further human and animal studies will need to be undertaken to prove this transgenerational inheritance hypothesis.
In that sense, some recent mouse studies suggest a mechanistic role for sperm miRNAs in the transgenerational transmission of paternal traumatic experiences [77,79]. Gapp and colleagues found that early traumatic stress induced remodeling of sperm miRNA expression in F0 but not F1 sperm. Notwithstanding, the behavioral and metabolic phenotypes were detected into the second (F2) generation [78]. On the other hand, Rodgers and colleagues discovered nine miRNAs (miR-29c, miR-30a, miR-30c, miR-32, miR-193-5p, miR-204, miR-375, miR-532-3p, and miR-698) that were altered in paternal sperm after exposure to chronic stress. Additionally, these miRNAs promoted and altered stress responses of the offspring [79]. Finally, in a very recent study, multiple miRNAs of the miR-449/34 family were differentially expressed in mice and men exposed to adolescent chronic social instability stress [77].
Taken together, these studies provide some evidence for the idea that miRNAs and DNA methylation markers contribute to the transmission of traumatic experiences, and therefore psychological stress, in mammals.
4.4.2.2 Air Quality and Pollution
The evidence that semen quality has been decreasing during the last 50 years is today very strong. It is also a fact that this decrease is stronger in certain geographic regions of the world, specifically in the developed and industrialized countries [41,80].
Currently there is some evidence that indicates that air quality and pollution could alter sperm parameters and result in male infertility [81,83]. However, to the best of our knowledge, only one study examined the relationship between air pollution and sperm epigenetics. In that study, the sperm DNA methylation was globally analyzed in 607 male partners of pregnant women from a large cohort and the authors found a significant association between exposure to persistent organic pollutants and a global decrease in sperm methylation [84]. With only this study it seems evident that further research needs to examine more closely the links between air quality and pollution and sperm epigenome.
4.4.2.3 Diet
Several observational studies suggest that adhering to a healthy diet (e.g. the Mediterranean diet) may improve male sperm quality parameters [85,86]. Also, a recent meta-analysis of randomized clinical trials (RCTs) proposes that some dietary supplements (e.g. omega-3, CoQ10, selenium, zinc, and carnitines) could beneficially modulate sperm quality parameters and affect male fertility [87]. However, results must be cautiously interpreted due to the limited sample size of the meta-analyzed studies and the considerable observed inter-study heterogeneity.
Presently only a few studies assessing the impact of diet on the sperm epigenetic signature have been published, therefore limited clinical conclusions or recommendations can be drawn. The effect of some nutrients, like folic acid and vitamin D, were analyzed in four different studies in mammals. First, Lambrot and colleagues identified multiple regions of the mouse sperm epigenome that are environmentally programmed. They discovered that, compared with folate sufficient males, mice receiving a folate deficient diet displayed alteration of sperm DNA methylation at genes implicated in development and some metabolic processes. Moreover, they have also demonstrated that a folate deficient diet is associated with negative pregnancy outcomes [88]. In humans, to the contrary of the aforementioned study, the administration of a high dose of folic acid (up to 10 times the daily recommended dose) daily, for 6 months, has been shown to also alter the sperm methylome. The general loss of methylation detected in this study was influenced by the presence of the MTHFR C677T polymorphism [89,90]. However, other authors have observed no effect on human sperm DNA methylation with the use of short-term (400 μg/day for 90 days) and long-term exposures to low-dose folic acid supplementation [91]. The only study testing the effect of vitamin D on the sperm epigenome revealed that depletion of vitamin D in mice resulted in more than 15,000 differentially methylated loci, and that most of these showed a loss of methylation. These differentially methylated CpGs were mainly localized in regions enriched for developmental and metabolic genes and pathways [92].
To the best of our knowledge, the only foods that have been tested in an RCT were nuts (walnuts, hazelnuts, and almonds). Healthy individuals consuming 60 g/d of raw nuts in the context of a Western-style diet during a period of 14 weeks improved various sperm quality parameters, but nut supplementation did not demonstrate any effect on global sperm DNA methylation. However, a significant reduction in hsa-miR-34b-3p expression levels in the group of individuals supplemented with nuts compared to the control group has been reported [93]. These data suggest that the sperm epigenome can be modulated by diet supplementation with certain nutrients and food. Nevertheless, due to the small number of studies published to date, further research may lead to a better understanding of the relationship between diet and the sperm epigenome.
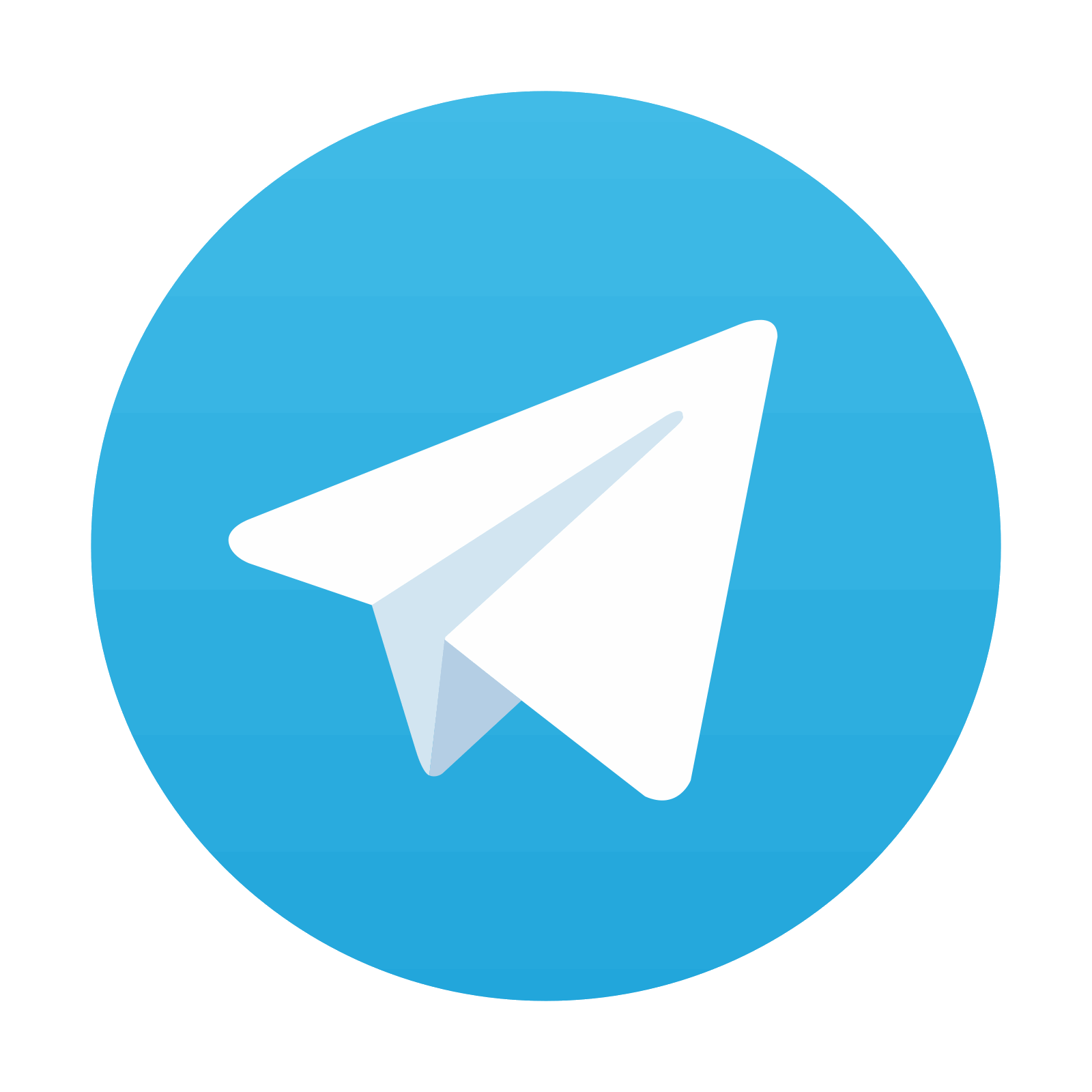
Stay updated, free articles. Join our Telegram channel
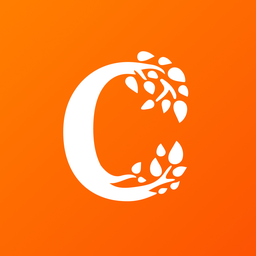
Full access? Get Clinical Tree
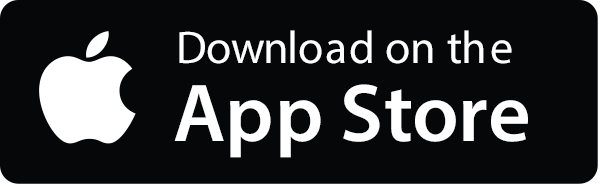
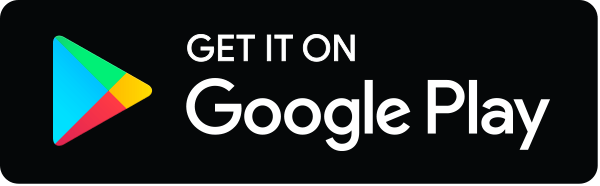