Abstract
Genetic risk is defined as the probability of a person (or couple) conceiving a pregnancy with genetic mutations or chromosomal abnormalities that would lead to a severe pathological condition. Genetic mutations are concealed in the genome of almost every person. Most of these mutations are harmless in single copy, but if present in homozygosis, they can lead to severe clinical outcomes. Often, couples carrying unidentified genetic mutations become aware of their dangerous mutations only after conception of an affected child.
Assessment of the Genetic Risk of a Couple Aiming to Conceive
Genetic risk is defined as the probability of a person (or couple) conceiving a pregnancy with genetic mutations or chromosomal abnormalities that would lead to a severe pathological condition. Genetic mutations are concealed in the genome of almost every person. Most of these mutations are harmless in single copy, but if present in homozygosis, they can lead to severe clinical outcomes. Often, couples carrying unidentified genetic mutations become aware of their dangerous mutations only after conception of an affected child. Around 1300 known recessive genetic conditions have been cataloged to date, affecting over 3 children in every 1000 and accounting for 20% of infant mortality and approximately 10% of pediatric hospitalizations in developed countries[1]. The 20 most common recessive genetic conditions are present in 1–2% of couples of reproductive age, who are thus exposed to a high risk of having a child affected by an inheritable genetic condition.
Total genetic risk of an individual encompasses inheritable conditions, such as mutations or certain chromosomal abnormalities (e.g., Robertsonian translocations), and genetic conditions, whose incidence is not hereditary but age-related (e.g., oocyte chromosomal aneuploidy).
The assessment of genetic risk in prospective parents (partners or patient and donor) through expanded carrier screening (ECS) tests would inform them of the likelihood of transmitting a genetic condition to their offspring. With this knowledge, alternative reproductive/preconception strategies (e.g., IVF+PGT) could be employed to avoid the passing on of a serious genetic condition. Additionally, for conditions where therapeutic interventions are possible, knowledge of carrier status may also allow for preventive and/or timely treatment/intervention, thus reducing morbidity and mortality in affected children.
Single-gene disorders include single-base mutations and triplet expansion genetic abnormalities associated with a pathogenic phenotype. Single-base mutations are commonly referred to as Mendelian traits as their inheritance pattern is completely controlled by one genetic locus, which follows recessive or dominant inheritance (whether autosomal or X-linked). In case of recessivity, the pathology is manifest when both copies of the gene carry the mutation, while in the case of dominance, a single mutated copy is sufficient to generate a pathological condition. An individual is usually aware of carrying a dominant mutation (except for de novo cases or low penetrance conditions) because it is likely to have been previously diagnosed in a family member. However, some dominant mutations (e.g., repeat expansion in dynamic disorders) are not manifest until later in life and direct inheritance may be unclear at the time of conception. These conditions are characterized by expansion and anticipation effect, which entail an increased length of the genetic expansion from one generation to the next, resulting in an earlier onset of the pathology. Similar to trinucleotide repeat disorders, other dominant mutations are not fully penetrant and the link between the presence of the mutation and their actual risk of pathological phenotype might be unpredictable (e.g., neurofibromatosis, NF1). Due to its clear manifestation across family members, mutations characterized by dominant inheritance will be investigated through family history evaluation by a genetic counselor. On the other hand, due to their uncertain pattern of manifestation, late onset and variable penetrance inheritance conditions are not within the scope of reproductive preconception screening and should also be investigated separately with the support of a professional geneticist. The possibility of performing ECS tests on prospective parents (or patient and donor), investigating for the presence of autosomal and X-linked recessive (AR, XLR) mutations clearly linked to pathological conditions, would clarify their genetic risk, enabling an informed choice on the reproductive strategy to undertake.
Certain inheritable conditions are not determined by the presence of a single mutation in a gene but by a combination of genetic and environmental factors that interact in ways not yet fully understood (e.g., type 2 diabetes, Alzheimer’s disease, and cancer). The genetic risk associated with a multifactorial condition cannot be precisely estimated as it depends on factors that are not coded in the DNA. However, the presence of certain mutations has been found to be highly associated with an increased risk of developing specific types of cancers (e.g., BRCA 1 and 2 for breast and ovarian cancer). Other genetic mutations involved in multifactorial conditions have been found to give a lower degree of predisposition to the pathology (e.g., BARD1 and BRIP1 for breast and ovarian cancer). Large studies evaluating genome-wide associations (GWAs) are being conducted to improve our understanding of the genetic basis of pathologies and precisely assess the relationship between genomic variants sets and the onset of several pathologies. Regression analysis of these data allows the calculation of polygenic risk scores, which serve as best approximation between the presence of defined multigene variants and the likelihood of developing a specific pathology. Currently, carrier screening testing for multifactorial conditions may not have definitive conclusions regarding the actual risk of onset of a polygenic condition in the offspring, but it may inform on specific predispositions allowing for preventive diagnosis and care. This information is currently given during genetic counseling and family history evaluation consultation sessions.
Most chromosomal abnormalities are incompatible with life, but a small minority of them lead to normal development and lifespan (e.g., balanced Robertsonian translocations), while others, although life-compatible, have appreciable effects on the individual’s phenotype (e.g., sexual chromosomes imbalances). Because of their compatibility with life, some aneuploidies may be present in the karyotype of individuals in reproductive age and therefore be transmitted to their offspring. The presence of a balanced or unbalanced translocation in the oocyte or spermatozoon that will form the embryo will determine embryo and fetus’ karyotype (normal or abnormal) for that chromosome. The ability to evaluate the presence of structural rearrangements in individuals looking to conceive a baby would determine whether they require ART (and screening of the specific condition in embryos generated through IVF) or if they are not at risk for that determined condition. In this case, the use of ARTs will drastically reduce the chance of miscarriage, fetal death, abnormal conception, and birth defects.
Age-related chromosomal conditions are not inheritable. They are caused by defects in the chromosomal set of germinal cells due to various reasons. The most common one is the accumulation of errors in the meiotic machinery, which results in a significantly inefficient process of germinal cell maturation during meiosis. This defective process is significantly more common in females than males due to the lengthy oocyte maturation progression[2]. According to standard scientific evidence, the number of female germinal cells is determined at birth and from this point in time they suspend their maturational process at an early stage of the first meiosis (dictyotene), which resumes during ovulation. There is solid evidence that the incidence of aneuploidy in embryos generated by women of advanced maternal age is significantly higher than in younger patients. This suggests that the longer the oocytes remain in this suspended maturational state, the higher the chance that the meiotic machinery accumulates defects and is unable to complete the process without resulting in the formation of chromosomal abnormalities. This defective process impacts the ability of females of advanced maternal age to naturally conceive a healthy baby. Since the occurrence of these chromosomal defects is not hereditary, ECS programs cannot precisely identify the risk of an individual generating chromosomally abnormal gametes or embryos. The only effective strategies to assess the presence of nonhereditary chromosomal abnormalities in embryos and fetuses remain preimplantation and prenatal genetic testing. Nonetheless, considerable variability in embryo aneuploidy rates has been reported across women of similar age undergoing preimplantation genetic testing for aneuploidy (PGT-A) cycles. It is therefore possible that part of the genetic determinants of aneuploidy risk may be unraveled in future studies, thus allowing specific risk assessment prior to conception.
Carrier screening platforms have been employed clinically for a few years using different technologies. However, common shortcomings have been the limited knowledge on infrequent genetic diseases and costs associated with multigene testing. A recent study by Haque and colleagues showed that incidence of severe genetic disorders in fetuses is greatly dependent on the parents’ ethnic background, with estimated risks ranging between 1:1000 (Hispanic) and 1:250 (Ashkenazi Jewish)[3]. The same study also demonstrated that current ECS panels show significant differences in diagnostic efficacy depending on the ethnical background of the population tested due to a skewed representation of ethnicity-specific mutations[3]. Although possible from a technical point of view, deep multigene assessment has been focused only on the most common pathological conditions due to the high analytical costs associated with parallel multigene analysis. However, this approach left certain ethnicity-specific conditions unscreened, resulting in lower test reliability for certain ethnic groups. Next generation sequencing (NGS) offers the possibility of expanding the breadth of preconception carrier screening capabilities to include a larger number of tested conditions and an increased number of variants investigated, resulting in a more comprehensive diagnostic tool compared to early ethnic-specific carrier screening platforms[4]. Nonetheless, significant differences in the number of conditions tested and their characteristics (e.g., inheritance pathway, severity, penetration, age of onset) and the degree of variant screening efficacy are present in currently available ECS strategies.
As a result, to guarantee an effective preventive strategy for Mendelian disorders at the pre-reproductive stage, careful analysis of the appropriate conditions to be included in the ECS panel should be expertly conducted and diagnostic benchmarks established[5]. In a well-designed ECS gene panel primarily targeting early-onset severe diseases, analytical validity of the tests should be properly investigated to ensure the information generated is reliable in detecting the presence or absence of specific genetic variants. Additionally, clinical validity of variants included in the test should be determined so that presence, absence, or predisposition to the related pathology is clearly established[6].
Therefore, by targeting several areas in the genome, NGS extends coverage of the screening test, drastically reducing residual risk. In this case, testing coverage is defined as the percentage of genetic risk detectable for AR and XLR conditions with an ECS platform. Inversely, residual risk in ECS is defined as the remaining inherent risk after removing the diagnostic information provided by the test.
Although NGS significantly increases the amount of information retrieved to about hundreds or thousands of genes, there are several limitations associated with this approach. For example, NGS may produce false-positive and false-negative findings. Additionally, by producing a detailed map of the whole coding genome when ECS is performed by exome sequencing (ES), much of the data generated may not be presently or unequivocally linked to specific phenotypes or pathological conditions. Moreover, clinical significance of certain complex variants may be uncertain and their association to a pathological phenotype difficult to evaluate, thus impacting on overall clinical validity of the test. For this reason, the characteristics of conditions tested and the scope of the test itself should be carefully considered and expertly reasoned to establish a rational benchmark for providers and patients. With current technology, effective ECS panels can be designed to (1) use systematic principles to include conditions with high prevalence/severity, (2) report pathogenic variants only, and (3) perform with high accuracy[6]. Additionally, growing use of exome/genome sequencing will reveal carrier frequencies for almost every disorder in the tested population, thus improving screening precision and meaningful residual risks calculation.
As discussed above, every person carries a number of mutations that could have drastic effects on the health of their offspring. The implementation of routine ECS is crucial for obtaining a reliable view on each individual genetic risk, allowing patients to make truly informed decisions about their reproductive life. Indeed, serious genetic conditions are recurrently found in the offspring of healthy parents. Studies on the application of ECS testing have revealed that the cumulative risk for healthy individuals to pass on a pathogenic mutation to their children is comparable to the risk for Down’s syndrome in the general population[3]. It is therefore possible to anticipate that an increasing number of the population will undergo ECS testing to receive information regarding their genetic risk. However, despite its enormous potential in improving reproductive and postnatal health, ECS platforms still suffer from both technical and diagnostic limitations, which will need exhaustive explanation to prospective patients prior to consenting for testing. In this regard, patients should receive detailed explanation regarding the benefits, risks, and limitations of ECS testing, so that they can make an informed decision on whether to submit to the test. Patients should also be able to decide the level of information they require and whether to include potential late onset conditions (e.g., cancer), low-degree of predisposition to certain pathologies[4].
Additionally, concerns regarding the release of personal genetic information retrieved using ECS to governments or insurers should be leveled by ensuring through legislation (e.g., the Genetic Information Nondiscrimination Act in the United States) that genetic information can be used only in a medical context, whether to prevent, treat, or avoid a pathological condition, but never to discriminate against individuals from a financial or legal standpoint.
As technology develops, it will be possible to assemble ECS panels that test for the most serious and common genetic conditions, leaving minimal residual risk. With parallel reduction in cost per analysis, ECS testing should become more affordable for patients favoring an extensive implementation in clinical routine. Additionally, ongoing GWA studies will likely improve overall clinical utility of ECS testing further progressing prediction, prevention, and treatment of an increasing number of genetic conditions. Similar to other forms of preventive screening, the ECS panel is likely to become a crucial tool for pathological investigation and treatment design. With the evolution of pharmacogenomics, ECS data will provide key information for enabling true personalized medicine, including administration of most effective drugs and dosages, predicting drug response or toxicity, and avoiding the application of ineffective or suboptimal treatment regimens. Notably, a single whole exome sequencing analysis would enable personalized medical intervention for the whole life by allowing retrospective evaluation of the sequencing data against most up-to-date genomic findings.
Assessing Endometrial Receptivity Using Prediction Models
The endometrial factor plays a key role in the reproductive process as a receptive endometrium is necessary for embryo implantation. The limited period in which the embryo can attach to the maternal endometrium is known as the window of implantation (WOI). Identifying an individual’s WOI allows for the synchronization of endometrial receptivity with an embryo ready to implant in a personalized embryo transfer (pET). This strategy improves outcomes of assisted reproduction for women with recurrent implantation failure (RIF)[7].
Commonly, histological criteria have been employed to date the endometrium. However, morphological criteria have major limitations in predicting endometrial receptivity, as demonstrated in randomized studies[8]. Endometrial receptivity has also been unsuccessfully investigated using several single markers[9].
Over the last decade, transcriptomics has emerged as a powerful tool in diagnosing disease and determining prognosis. The reproductive field is not an exception and the transcriptomics of the human endometrium has been widely investigated[10]. The main interest has focused on the identification of the specific transcriptomic signature that can identify a receptive endometrial function and improve the effectiveness of reproductive treatments.
Endometrial receptivity analysis (ERA) was the first transcriptomic test introducing the concept of pET by timing ET according to each patient’s personalized WOI (pWOI) [7,11]. Although most women reach receptivity after five full days of progesterone administration, some of them are affected by WOI displacement. Application of ERA revealed that 25% of patients experiencing RIF had a displaced WOI, with a faster or slower response to progesterone, leading to asynchrony between embryo and endometrium. Therefore, it is very important to identify a specific WOI for each patient to synchronize the transfer of a viable blastocyst with a receptive endometrium. ERA testing has been clinically applied since 2010, and different groups around the world have reported its clinical results[12,13].
ERA was initially developed as a microarray-based clinical test measuring the transcriptomic signature of 238 genes to assess endometrium receptive status[11]. This customized microarray was coupled with a computational predictor able to identify the specific transcriptomic profile for each endometrial stage, namely proliferative (PRO), pre-receptive (PRE), receptive (R), and post-receptive (POST).
Recently, an increasing number of researchers are choosing NGS over microarray for transcriptomics investigations. The use of a sequencing technology that delivers fast, cost accessible, and accurate transcriptomic information has motivated the chapter authors’ team to move from microarray-based ERA to NGS-based ERA 2.0.
ERA 2.0 is a predictor tool based on a machine-learning classification model[14]. Classification models are supervised learning methods for predicting the value of a categorical target attribute. Models are developed using a set of past observations whose target class is known and a set of explanatory variables. A prediction (class probability) is obtained by applying the model to new samples.
Specifically, the explanatory variables are the ERA genes counts obtained by sequencing files and the categorical attribute to predict is the different endometrial receptivity profiles. In summary, our objective was to identify the different endometrial stages using the transcriptomic profile determined by the original ERA genes. The identified profile gives indications on how to adjust progesterone administration to reach optimal endometrial receptivity: pre-receptive requiring 2 extra days (PREd2), pre-receptive requiring 1 extra day (PREd1), early receptive requiring 12 extra hours (ER), R, late receptive requiring 12 hours less (LR), POST requiring 1 day less, or PRO, which would imply absence of progesterone in the endometrium or a lack in response to it.
In a classification task, it is very important to select well-defined and curated samples to train the predictor model. This was warranted by the chapter authors’ expertise acquired through the analysis of over 20 000 endometrial samples worldwide.
The data set for NGS predictor development comprised 411 endometrial biopsies in which ERA was previously diagnosed by microarray technology. This data covered all the different profiles: PREd2, PREd1, ER, R, LR, POST, and PRO. The receptive group included samples with successful pregnancy after pET, while the nonreceptive group comprised samples with a second ERA biopsy reaching receptivity after WOI correction based on first ERA recommendation. This dataset comprised women with a mean age of 37.5±5 years old (mean +/- standard deviation), with BMI mean of 23.3±3.6 Kg/m2. Most of the samples were taken in hormone replacement therapy (HRT) cycles (82%), while the remaining were taken in natural cycles.
These data were randomly split into training set (70% of data set) and test set (30% of data set). The training set was used for building the classification model (n=290 samples), and the test set (n=121) was used to provide an unbiased performance evaluation.
During algorithm development, several models were studied to find those most appropriate for our data. Four of the most popular machine-learning algorithm methods for classification problems were compared: support vector machine, k-nearest neighbor, random forest (RF), and classification tree[15]. Optimal tuning parameters for each model were selected based on a resampling technique (a repeated k-fold cross-validation).
The performance of each model was computed over the test set in terms of global accuracy, sensitivity, and specificity. After comparing all models, the RF obtained the best results with an accuracy of 0.88 (95% CI 0.80–0.92). As we had more than two classes, the performance was calculated from “one-versus-all”; the mean sensitivity was 0.90 and mean specificity was 0.97.
The predictor identified when the endometrium reached receptivity during the cycle. So, if a first ERA was done at the expected day of WOI (progesterone (P) + 5, luteinizing hormone (LH) + 7, or human chorionic gonadotropin (hCG) + 7) with a nonreceptive result, a second endometrial biopsy was recommended on the day predicted by ERA 2.0.
An analysis was performed using paired-biopsies verification compound by 1 432 endometrial samples (n=716 paired samples). The first biopsy of these paired biopsies was predicted to need one more day of progesterone administration (diagnosed as PREd1). A successful match was considered when the patient followed the ERA recommendation and the result of the second biopsy was R, or if the patient did not follow the ERA recommendation and the result was nonreceptive (NR) (as expected).
The paired biopsy verification analysis was evaluated in terms of percentage of prediction success after a second biopsy. Out of the 716 PREd1 paired samples, the success proportion was 0.89 (95% CI 0.86–0.91).
Figure 2.1 shows the workflow followed to identify the endometrial receptivity by the ERA 2.0 test.
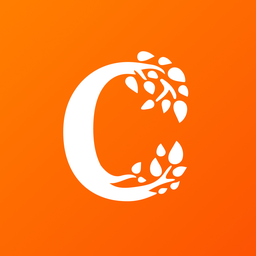
Full access? Get Clinical Tree
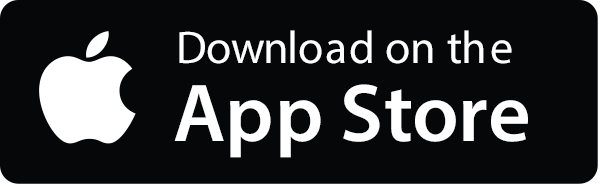
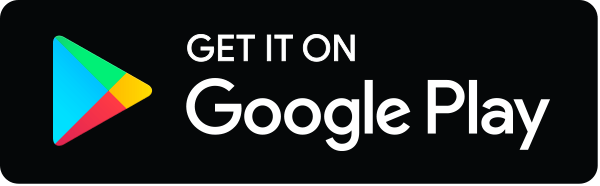