Abstract
All sperm accrue varying amounts of DNA damage during maturation and storage, a process that appears to be mediated through oxidative stress. The clinical significance of genetic damage in the male germ line depends upon severity and how that damage is distributed among the sperm population. In human reproduction, the embryo is capable of significant DNA repair, which occurs prior to the first cleavage event. However, when the magnitude of genomic damage reaches pathologic levels, reproductive outcomes begin to be affected. Evidence now exists linking excessive sperm DNA fragmentation with time to pregnancy for natural conception, pregnancy outcomes of intrauterine insemination and in vitro fertilization, and miscarriage rates when intracytoplasmic sperm injection is employed. This review will discuss the pathophysiology of sperm DNA damage, the studies linking it to impaired reproductive outcomes, and how clinicians may render treatment to optimize the chance of paternity for their patients.
17.1 Introduction
DNA damage is a common feature of all spermatozoa. The relative magnitude and clinical meaningfulness of sperm genomic integrity varies from cell to cell, with each man producing a population of spermatozoa that will carry a unique distribution of DNA damage. Additionally, this distribution will vary with time, sperm source, and the lifestyle/exposures that occur during the period of spermatogenesis. Given the ubiquity of genomic lesions that occur in the male germ line, it is apparent that the embryo is capable of robust DNA repair with high fidelity. However, the embryo’s ability to compensate for defects in the paternal genome does have limitations, which is apparent in data correlating male subfertility with progressively higher levels of DNA fragmentation. These observations are apparent in natural conception rates and time to pregnancy studies, a trend that has also translated to outcomes of assisted reproductive technologies (ART). Despite these data, reproductive medicine has been slow to adopt sperm DNA testing due to the lack of assay standardization, controversy regarding normal thresholds, and a body of literature that suffers from significant design/cohort heterogeneity with equally heterogeneous results. In the following review, the pathophysiology of sperm DNA damage will be discussed along with a description of the sequalae that can be expected. Finally, the data associating DNA fragmentation in sperm with reproductive outcomes will be outlined, including therapeutic options currently available for men faced with significant levels of sperm DNA damage.
17.2 Genomic Integrity
17.2.1 Categorization of Genetic Damage
The unique process of spermatogenesis exposes the male germ line to a specific set of genetic insults that are not typically encountered by somatic cells. Relevant hallmarks of spermatogenesis include the life-long maintenance of a stem-cell population, differentiation, meiosis with genetic recombination, successful production of a haploid cell line, and ultimate maturation into functional spermatozoa. The success of spermatogenesis is also dependent upon appropriate support and surveillance by surrounding Sertoli cells, the creation of a suitable paracrine and endocrine milieu, and the careful regulation of testis temperature. Impaired function at any of these levels can result in male subfertility. In terms of genomic integrity, aberrant function of the aforementioned processes can directly or indirectly affect the viability of the sperm product. Regardless of the cause, the majority of genetic damage can be categorized into three non-mutually exclusive endpoints: chromosomal abnormalities, errors in DNA packaging, and DNA fragmentation.
Chromosomal abnormalities include errors of chromosome number, deletions, and transversions. The majority of these types of genetic faults are associated with errors in meiosis. Overall, the testis is efficient in recognizing cells that have suffered an error of meiosis, and typically eliminates them prior to maturation into functional sperm. In men with proven fertility, the rate of aneuploidy is low in ejaculated specimens, with rates ranging below 1% [1]. The proportion of sperm with abnormal ploidy is elevated in both men with impaired semen parameters and in men who contribute to recurrent pregnancy loss with otherwise reassuring semen analyses [2]. The assay used to detect sperm aneuploidy has conventionally been fluorescent in situ hybridization with probes designed against specific chromosomes of interest (typically the sex chromosomes with the addition of 13, 18, and 21 [1]).
The second category of genetic damage encompasses errors in DNA packaging. Unlike somatic cells that carry their genetic material around histones, the majority of sperm DNA is compacted with protamines. The tertiary structure of protamines is approximately half the size of histones, but compacts the genome to approximately one-sixth the size observed in somatic cells [3]. Beyond size considerations, the function of protamination also engenders the genome with a significant level of protection from attack by reactive oxygen species (ROS) [4]. To achieve the level of compaction characterized by protamination, the DNA backbone becomes progressively supercoiled during rewinding around these protein structures. To limit torsional stress, topoisomerases cause temporary physiologic nicks in the DNA [5]. These DNA breaks allow for relief of torsional stress and are normally repaired without consequence. However, defective repair of these DNA nicks can lead to persistent DNA double-strand breaks in mature sperm, one possible mechanism of DNA fragmentation [6]. Although there are no widely utilized assays to measure protamination clinically, defective chromatin compaction has been linked to subfertility in both animal models and humans [7.8].
The third category of DNA damage is typically termed “DNA fragmentation,” since it is measured by direct or indirect assays of DNA strand breaks (Table 17.1). However, the range of DNA damage that may ultimately result in fragmentation is broad. Table 17.2 provides a brief list of the types of DNA damage that may occur in spermatozoa. Although grouping these distinct processes under the DNA fragmentation header is imprecise, no widely used tests exist to assay each specific type of genetic insult in clinical practice. In lieu of mechanism-specific damage measurement, the clinician must therefore rely upon the global assessment of DNA fragmentation to provide an impression of genomic integrity.
Assay | Fragmentation assessment | Chemistry | Application |
---|---|---|---|
COMET | Direct | DNA with single and double strand breaks will migrate during gel electrophoresis, producing a tail stemming from the sperm nucleus | Capable of assessing DNA damage of individual cells. Requires a fresh semen sample. Time and labor intensive. Lacks well established protocols. |
TUNEL | Direct | Exposed single and double strand DNA nicks are labeled with fluorescent dUTP with a template independent deoxynucleotidyl transferase | Commercially available kits. Can be conducted on fresh or frozen specimens. Also lacks established protocols and standardized thresholds. |
SCSA | Indirect | Acridine orange fluoresces at differing wavelengths for intact vs denatured DNA. DNA with fragmentation will denature more readily and produce more signal | Frozen specimens are mailed to a commercial lab. Requires samples with large numbers of spermatozoa. Benefits from established clinical thresholds. |
SCD | Indirect | Single cells are embedded in agarose gel, lysed, and the DNA is denatured. Undamaged DNA will produce a “halo” around the nucleus | Inexpensive commercial assays can be purchased that do not require fluorescent imaging. Noisy/unclear cells may be misinterpreted. |
TUNEL: Terminal deoxynucleotidyl transferase-mediated dUTP nick end labeling. SCSA: Sperm chromatin structure assay. SCD (also known as “halo”): Sperm chromatin dispersion assay.
Table 17.2 Types of DNA damage possible in the sperm nucleus.
Types of DNA damage |
---|
Base adducts |
Abasic sites |
Guanosine oxidation |
Pyrimidine dimerization |
Thymidine glycol |
Base modifications |
Crosslinks |
Mismatches |
These types of genetic lesions are not directly measured by DNA fragmentation assays.
Unlike errors of meiosis, some level of DNA fragmentation is thought to exist in every sperm, with the relative degree of genetic damage varying from cell to cell [9,10]. As a result, depending upon the type and severity of genomic damage, viable embryos will undergo a round of DNA repair prior to the first cleavage [6]. Although the embryo is well equipped to address this type of damage, viability will ultimately hinge upon the quality and quantity of DNA damage. A variety of risk factors have been linked to DNA fragmentation (Figure 17.1). Despite the myriad of causes, many investigators have effectively argued that oxidative stress forms a common mechanistic pathway [6,11,12]. Given the ubiquitous nature of DNA fragmentation in all males, the remainder of this chapter will concentrate on the pathophysiology, clinical consequences, and treatment of this type of genetic damage.
17.2.2 Pathophysiology of DNA Fragmentation
DNA fragmentation has been linked to an array of male infertility risk factors, including widely disparate conditions such as age, varicocele, cigarette smoking, and infection/inflammation. The pervasive endpoint of genomic instability would suggest a common mechanistic pathway for the conditions outlined in Figure 17.1. Seminal work by De Iuliis and colleagues explored the correlation of oxidative damage with a specific base adduct, 8-hydroxy-2′-deoxyguanosine (8OHdG), to the level of DNA fragmentation [4]. 8OHdG is a type of DNA modification that occurs following a direct oxidative insult of the DNA molecule itself, and has traditionally served as a robust biomarker of oxidative stress. The abundance of 8OHdG residues correlated so well with the amount of DNA fragmentation that some investigators “have been forced to conclude that most DNA damage in spermatozoa is oxidatively induced” [4,6]. It is worth mentioning that in correlated studies most of the risk factors listed in Figure 17.1 were also tied to an excessive oxidative state [13].
The role of ROS in male fertility is complex, with evidence showing that a mild oxidative state is necessary for appropriate sperm function. Early studies demonstrated improvement of fertilizing capability of human spermatozoa subjected to low levels of oxidative stress [14]. It is now appreciated that ROS are required for effective capacitation of sperm in multiple species, including humans [13,15]. The presence of ROS increases intracellular cAMP, inhibits tyrosine phosphatases, and promotes the oxidation of sterols in the spermatozoon head, all of which are necessary for effective capacitation [11]. The process may be initiated by either endogenous or exogenous ROS. H2O2 appears to be a key element, as the addition of catalase can prevent hyperactivation, membrane fusion, and the acrosome reaction [15]. The balance between ROS and antioxidants is critical however, as too much oxidative stress will result in DNA damage and possibly initiate apoptosis. To this end, it has been observed that morphologically normal sperm with hyperactive motility can harbor significant genomic damage, yet they can still perform well in terms of the ability to fertilize [16]. It is conceivable that sperm primed for capacitation, which may have already accrued pathologic levels of DNA fragmentation, will outperform sperm with less DNA damage as a function of ROS exposure.
As oxidative stress increases, the apparent continuum shifts away from the physiologic state described above and switches toward a form of apoptosis. Nearly all sperm are ultimately destined to either undergo apoptosis or senescence, as only a few will actually participate in fertilization. The unique process of sperm cell death is significant, as these cells must be consumed by phagocytic leukocytes in both the male and female reproductive tract without triggering an inflammatory response. The silent removal of these cells is ensured by the display of apoptotic markers on dead sperm, the most important being phosphatidylserine [12]. Although this is a common feature of apoptosis in general, the process of apoptosis in spermatozoa deviates substantially from somatic cells.
Apoptosis is an active process that relies upon intact transcription, translation, the expression of FAS (exogenous pathway), and the co-compartmentalization of the nucleus and mitochondria (endogenous pathway). A characteristic feature of somatic apoptosis is the laddering of nuclear DNA on gel electrophoresis, which is a consequence of endonuclease fragmentation of the genome. However, apoptotic sperm do not exhibit this laddering pattern [12]. Additionally, sperm do not respond to the conventional chemical triggers of apoptosis [12]. To understand these fundamental differences and how they are important clinically, one must recognize the exceptional barriers to efficient apoptosis that exist in sperm.
Initially, the developing germ line is capable of traditional apoptosis. The number of maturing gametes is controlled by surrounding Sertoli cells, which express FAS-ligand and triggers the exogenous apoptosis pathway [9–12]. This population control ensures that Sertoli cells have enough resources to provide proper support. However, as spermatocytes advance toward spermatozoa, they progressively lose the ability for transcription and translation. Additionally, the replacement of histones with protamines during spermiogenesis impacts on how endonucleases react with DNA, since the primary endonuclease Caspase-Activated-DNase (CAD) usually acts upon the linker segment between histones [12]. As spermatids elongate, the cytosol and mitochondria become compartmentalized into the midpiece, effectively sequestering them from the nucleus located in the sperm head. As a result, much of the dormant CAD in the cytosol can no longer access the nuclear material.
Beyond ineffective endonuclease activity, the unique structure of the sperm carries additional implications for canonical apoptosis. The mitochondria are a keystone to the endogenous pathway of apoptosis, and similar to the discussion regarding CAD, in sperm they are also functionally sequestered from the nucleus. The mitochondria are potent producers of ROS, which will typically be activated by the absence of “survival” signals presented to the spermatozoon via the PI3 K-AKT pathway [12]. The mitochondria will also initiate the endogenous pathway if they sense significant cellular damage, mainly in the presence of lipid aldehydes or exogenously produced ROS. Regardless of the initial precipitator, once the mitochondria switch to an apoptotic mode a progressive cycle begins that leads to cellular death.
Mitochondria initially increase their production of ROS, which begin to react with cellular components necessary for sperm function. These reactive molecules tend to attack polyunsaturated fatty acids, which are particularly abundant in spermatozoa. A lipid peroxidation cascade begins that culminates in the formation of toxic lipid aldehydes [16]. Both free unsaturated fatty acids and the presence of lipid aldehydes provoke the mitochondria to release more ROS. The positive feedback loop that ensues generates a surge of oxidative damage. One of the first elements to be affected is motility, which is thought to be secondary to depletion of ATP. Another possible mechanism is the production of protein adducts within the axoneme machinery via mitochondrial derived ROS and reactive lipid aldehydes. It has been shown experimentally that the mere addition of lipid aldehydes, at physiologic concentration, will impair sperm motility [12]. Simultaneously, accumulating oxidative damage within the cell membrane impairs the ability of the sperm to fuse with an oocyte.
The positive feedback loop that results in the surge of endogenous oxidative stress is likely adaptive since spermatozoa cannot undergo full apoptosis. To remove themselves from the pool of viable spermatozoa, the cell must incapacitate both its fertilizing capability (plasma membrane and motility) and dismantle its genetic payload. Since sperm cannot dispose of its DNA with endonucleases, the only element in the system that can reach the genetic material is the ROS that are produced. The toxic metabolites, such as lipid aldehydes, in addition to direct oxidative damage of DNA, produce a variety of genetic defects, as presented in Table 17.2. With time, DNA fragmentation becomes a final common endpoint as profound damage ensues and the spermatozoon progress toward death. Unfortunately, a period will exist in which the sperm has begun the process of apoptosis, but remains capable of fertilization. This state has been loosely termed “abortive apoptosis,” whether it originates during maturation in the seminiferous tubules or in transit through the male or female genital tracts [5].
It is important for the fertility specialist to understand the process of sperm apoptosis and its link to genetic integrity. First, some amount of oxidative stress is needed to prime sperm for capacitation and fertilization. However, it is unclear when this balance may shift and moderation has been surpassed, potentially resulting in significantly damaged sperm outperforming unprimed cells with preserved genomes. Although motility is one of the first functions to diminish in the process, again the state of abortive apoptosis is misleading. Men with apparently “normal” semen parameters, motility being the most relevant variable, may be generating spermatozoa with moderate levels of DNA damage that is sufficient to affect reproductive outcomes. Finally, in terms of advanced reproductive technology, the selection of morphologically normal and motile sperm for ICSI is helpful, but not a guarantee, for selecting a cell that has minimal DNA damage. As a result, this latter concept may provide the basis for why ICSI seems to outperform IVF for men with significant levels of DNA fragmentation [17].
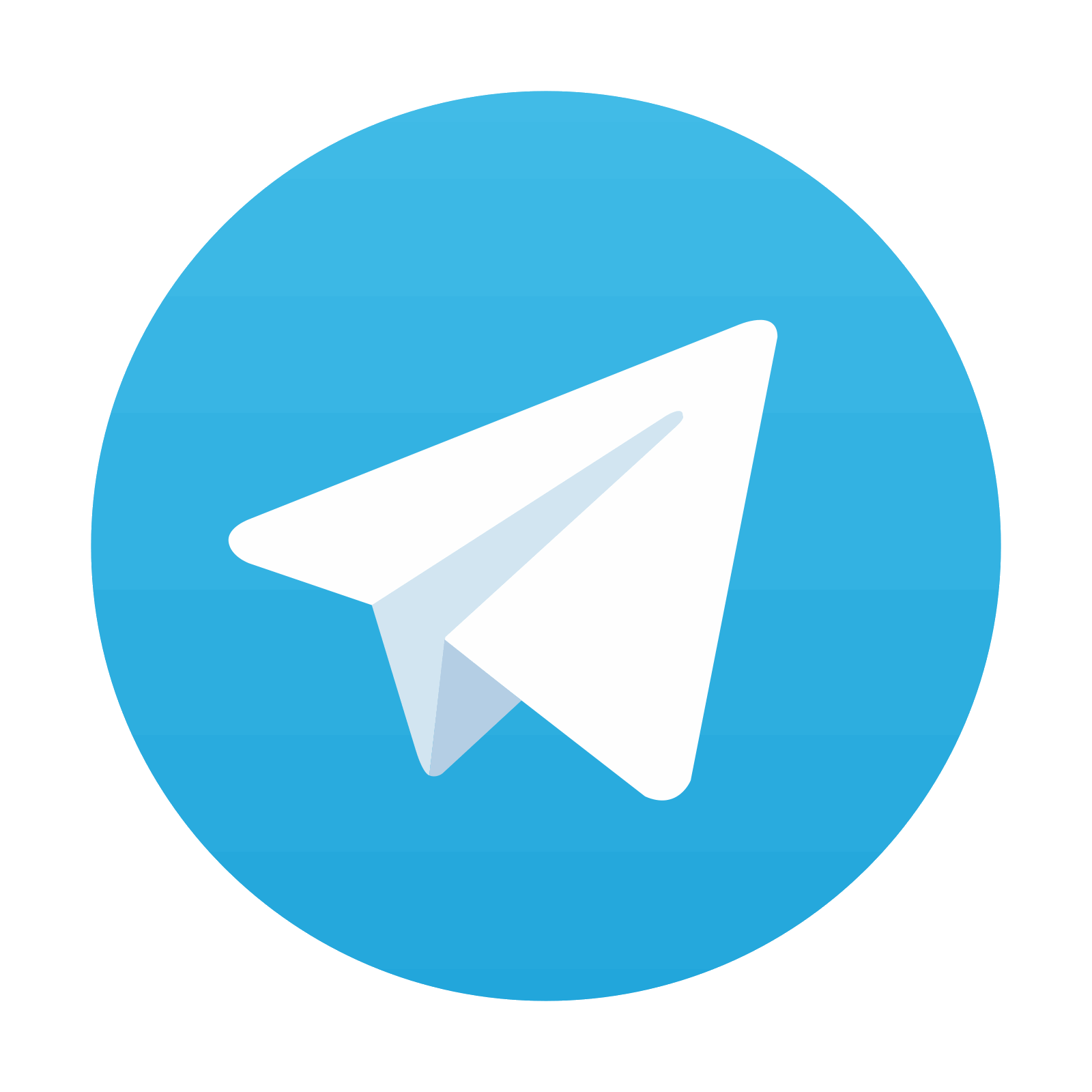
Stay updated, free articles. Join our Telegram channel
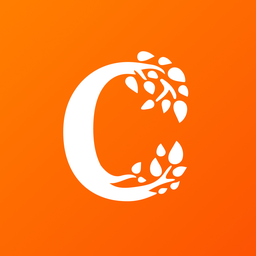
Full access? Get Clinical Tree
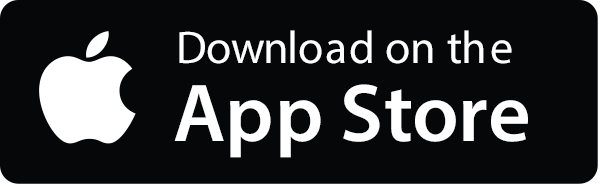
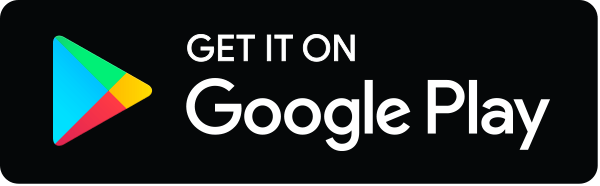