Abstract
Preimplantation genetic diagnosis (PGD) was developed in the late 1980s to help couples who are at risk of transmitting an inherited disease to their offspring, as an alternative to prenatal diagnosis during pregnancy. Prenatal diagnosis has the disadvantage that if the diagnosis shows the fetus to be affected, the couple must decide whether they wish to terminate the pregnancy or continue with the knowledge that their child is going to be affected by the genetic disease. PGD offers some of these couples an alternative, as the diagnosis is performed on the preimplantation embryo, and only embryos assessed as being unaffected by the genetic disease are transferred to the patient. The pregnancy is therefore initiated with the knowledge that the fetus is free from the disease, at that moment in time.
Introduction
Preimplantation genetic diagnosis (PGD) was developed in the late 1980s to help couples who are at risk of transmitting an inherited disease to their offspring, as an alternative to prenatal diagnosis during pregnancy. Prenatal diagnosis has the disadvantage that if the diagnosis shows the fetus to be affected, the couple must decide whether they wish to terminate the pregnancy or continue with the knowledge that their child is going to be affected by the genetic disease. PGD offers some of these couples an alternative, as the diagnosis is performed on the preimplantation embryo, and only embryos assessed as being unaffected by the genetic disease are transferred to the patient. The pregnancy is therefore initiated with the knowledge that the fetus is free from the disease, at that moment in time.
Patients referred for PGD include those who have already experienced several terminations of affected pregnancies or have experienced repeated miscarriages due to unbalanced chromosome arrangements in the fetus, those with moral or religious objections to termination, and infertility patients who carry a genetic or chromosomal abnormality.
The technology and expertise involved in PGD require the participation of, and close collaboration between, three separate disciplines: genetics, IVF and molecular biology.
Chromosomes, Genes and the Genetics of Inherited Disease
Human cells contain 23 pairs of chromosomes, 46 in total; each parent contributes one chromosome to each of these pairs. Twenty-two of the chromosome pairs, known as autosomes (chromosomes 1–22), have the same appearance in males and females; females have two X chromosomes as the 23rd pair, and males have one X and one Y chromosome as their 23rd pair. Reproductive cells (gametes) have 23 chromosomes, 22 autosomes and either one X (oocytes) or one Y (sperm cells) chromosome.
Autosomal genes are therefore present in two copies, one inherited from each parent; females have two gene copies on their X chromosomes, whereas males have only one copy of genes carried on their X and Y chromosomes. Genetically inherited diseases are usually caused by a mutation within a specific gene, which causes the gene to be inactive or faulty. The mutations that lead to a disease can be caused by a single change, or by more complicated changes within the gene, such as deletions, substitutions or insertions in the base sequence. A single gene may contain ‘hot spots’ that are prone to mutation. For example, although over 800 mutations have been identified in cystic fibrosis (CF), 70% of individuals in the United Kingdom who carry CF have the same mutation (deltaF508), caused by the deletion of three base pairs in exon 10 of the CF gene, which is located on chromosome 7.
A triplet of bases on a chromosome can sometimes be expanded, and this phenomenon leads to a group of diseases known as triplet repeat disorders. Gross chromosomal abnormalities, such as aneuploidy, translocations and inversions, can also lead to a fetus with an unbalanced chromosome complement. Age-related aneuploidy can also lead to chromosomally abnormal offspring: this is not an inherited disease, and it can occur in any pregnancy.
Single-gene defects may affect the autosomes (chromosomes 1–22) or the sex chromosomes (X and Y). Whether the disease is expressed when both or only a single copy of the gene carries a mutation is determined by the mode of inheritance: autosomal recessive, autosomal dominant or X-linked (sex-linked).
Autosomal Recessive Disease
Autosomal recessive inheritance accounts for the majority of genetic disease; the pattern of its inheritance is outlined in Figure 14.1. If an individual has one normal and one abnormal gene for a particular disorder, he or she is a carrier of the disease and will usually be unaffected. If both parents of a child carry the same disease-causing mutation, the child will be affected by the disease. For example, if both the mother and the father are carriers of cystic fibrosis, the offspring have a 1 in 4 chance of being affected, 1 in 4 chance of being unaffected, and a 2 in 4 chance of carrying the disease without being affected.
The most common autosomal recessive single-gene defect is beta-thalassemia, which is caused by a mutation in the beta-globin gene. However, there are many different mutations for beta-thalassemia, especially between different ethnic groups: in many couples, each partner may carry a different mutation (compound heterozygotes).
Cystic fibrosis was one of the first autosomal recessive single-gene defects to be diagnosed by PGD. Since deltaF508 is a relatively common mutation, it may be carried by both partners in many couples. Table 14.1 shows examples of major autosomal single-gene defects that have been diagnosed by PGD.
Autosomal Dominant Disorders
Disorders that are dominant in their inheritance will be expressed if a single copy of the mutated gene is present (Figure 14.2). These diseases are not as life-threatening as some recessive diseases, and therefore affected individuals can still reproduce and transmit the disease to their offspring. Many dominant disorders are late in onset, such as Huntington’s disease and some inherited cancers. A large number of dominant diseases can now be diagnosed by PGD, including myotonic dystrophy, Marfan’s syndrome, polyposis coli, Charcot–Marie–Tooth disease and Huntington’s disease (Table 14.2).
X-linked Diseases
X-linked diseases affect genes that are carried on the X chromosome: more than 400 X-linked diseases have been identified. They can be inherited in a recessive or dominant manner, but almost all severe types have recessive inheritance. Males inherit the X chromosome from their mother, and if this inherited X chromosome is abnormal, they will be affected with the disease (Figure 14.3). Therefore, carrier mothers transmit the disease to half of their male offspring, and half of a carrier mother’s daughters will be carriers. Embryo biopsy and genetic diagnosis can identify noncarrier female and nonaffected male embryos, which can then be selected for transfer. Some of the X-linked diseases reported as diagnosed by embryo sexing are listed in Table 14.3a, and Table 14.3b shows examples of X-linked diseases where a specific diagnosis has been performed.
Figure 14.3 X-linked inheritance.
Table 14.3 Examples of X-linked diseases diagnosed by PGD
Triplet Repeat Disorders
A new class of genetic disorders was identified during the 1990s: triplet repeat disorders are caused by the expansion of a triplet of bases that are repeated within a gene, and they are usually associated with neurological disorders. Each disease has a range of repeats associated with a spectrum from normal individual to affected individuals.
For example, fragile X syndrome, which generally affects males, was originally thought to be an X-linked disease, but it has been reclassified as a triplet repeat disorder. The disease is caused by the unstable expansion of a CGG repeat in the 5′-untranslated region of the FMR1 gene, which lies on the X chromosome. This triplet expansion results in mental retardation; a normal individual will have from 6 to 54 triplet repeats, those having the ‘premutation’ will carry between 54 and 200 repeats, and those affected with fragile X will have over 200 repeats. Females carrying the premutation are at risk of transmitting the full mutation to their offspring, and since males inherit the X chromosome from their mothers and have a single X chromosome, their male offspring are at a 50% risk of inheriting fragile X. Females who inherit the expanded gene from their mothers will also inherit a normal X chromosome from their father, and their disease manifestation is variable. Males carrying the premutation are at risk of transmitting only the premutation to their female offspring, who will be carrier females.
Huntington’s disease (HD) is a progressive neuropsychiatric disorder of late onset that is inherited in a dominant fashion. The gene is on chromosome 4 and involves a CAG triplet repeat where expansion beyond 36 results in HD. The age of onset is about 40 years and patients often die by their mid-50s. PGD has been performed for HD, but it draws some ethical discussion: many potential carriers of HD know that they have a 50% risk of being affected because one of their parents is affected, but they do not wish to know their own HD status. For prenatal testing and PGD, an exclusion test can be offered, where patients are given a risk factor without being told their actual status.
Myotonic dystrophy (DM) or Steinert’s disease is a progressive muscular dystrophy. The gene is located on chromosome 19, and DM is caused by expansion of a CTG repeat at the 3′-untranslated part of the myotonic dystrophy protein kinase gene. Normal individuals have between 5 and 37 repeats, and affected individuals may have anything from 50 to several thousand repeats. Intermediate numbers of repeats can give rise to a premutation. DM can be diagnosed by PGD.
Chromosomal Abnormalities
Abnormalities that involve whole chromosomes are usually lethal. Those compatible with life include Down’s syndrome (three copies of chromosome 21) and those that involve the sex chromosomes, such as Turner’s syndrome (XO) and Klinefelter’s syndrome (XXY).
The most common chromosome abnormality is a translocation, where two chromosomes have broken and rejoined to the opposite chromosome. If the chromosomes are still balanced, i.e., all the genetic material is still present, the patient is described as having a balanced translocation. The majority of patients carrying a balanced translocation do not realize they have abnormal chromosomes until they try to reproduce. During meiosis, the segregation of the chromosomes becomes confused, and unbalanced chromosome complements are formed in the gametes, leading to the formation of an embryo with abnormal chromosomes (unbalanced translocation). Therefore, patients carrying balanced translocations may experience infertility, repeated miscarriage or the birth of a child with abnormal chromosomes.
Robertsonian translocations involve breakages around the centromere of the ‘acrocentric’ chromosomes (13, 14, 15, 21, 22). These chromosomes contain a satellite region on their short arm, and loss of this area has no effect on phenotype. Since two of the acrocentric chromosomes join together, the patient has only 45 chromosomes. Robertsonian translocations can be diagnosed using PGD to identify the number of chromosomes present.
Reciprocal translocations involve breaks at any location on two chromosomes, and any chromosome can be affected. Every couple may have different chromosome breakpoints, and therefore diagnosis by PGD is difficult.
Chromosome abnormalities can also be caused by chromosome inversions, insertions, deletions or rearrangements (such as ring chromosomes).
Age-Related Aneuploidy
Women over the age of 35 are known to be at increased risk of having a fetus with a chromosome abnormality such as Down’s syndrome. However, only 20% of Down’s syndrome babies are born to women over the age of 35. Screening methods have been developed to help identify those pregnancies at risk, as the use of age alone as an indication for prenatal diagnosis of age-related aneuploidy will miss the majority of affected pregnancies. Biochemical (plasma alpha-fetoprotein, hCG, unconjugated estriol) and ultrasound screening methods are therefore used to determine which pregnancies are at risk. Patients found to be at risk undergo prenatal diagnosis, with a karyotype performed to ascertain the status of the fetus. The chromosomes most commonly involved in age-related aneuploidy are 13, 16, 18, 21, X and Y.
Prenatal Diagnosis
Aneuploidy Screening
Serum Screening
During the second trimester of pregnancy (16–17 weeks) a number of markers have been found that help to identify pregnancies with chromosome abnormalities. Down’s syndrome pregnancies show lower maternal serum alpha-fetoprotein (AFP) and unconjugated estriol, whereas human chorionic gonadotropin (hCG) levels are two times higher than normal. Taking into account the patient’s age, a triple screening test to measure AFP, free beta-hCG and unconjugated estriol can increase the detection rate to 70%, thereby reducing the number of women who require invasive prenatal diagnosis. Pregnancy-associated plasma protein A (PAPP A) is reduced in Down’s syndrome pregnancies. Noninvasive prenatal diagnosis tests (NIPD) that screen cell-free DNA in maternal serum are now also available (see below).
Ultrasound
During the first trimester of pregnancy, ultrasound detection of nuchal translucency measuring greater than 3 mm is associated with a chromosome abnormality. This is caused by fluid accumulation at the back of the fetal neck. In conjunction with maternal age, studies have shown this to give a detection rate of 86% with a false positive rate of 4.5%. When used in combination with first and second trimester serum screening, a detection rate of over 90% has been reported.
Around one-half of all major structural abnormalities can now be detected by ultrasound in the first trimester, including acrania/anencephaly, abdominal wall defects, holoprosencephaly and cystic hygromata. Other anomalies become evident later in the second trimester, as organ systems develop: cardiac malformations, duodenal atresia, hydrops, choroid plexus cysts, nuchal edema, renal pyelectasis, omphalocele, hypoplastic midphalanx of the fifth finger, and short femur and humerus can be used to screen for aneuploidy. Digital imaging combined with computer analysis of two-dimensional ultrasound pictures can be used to create three- and four-dimensional images, which allow more in-depth assessment of cardiac and neural abnormalities, and show a clearer picture of what specific abnormalities look like.
Diagnosis of Inherited Disorders
Couples who have already had an affected pregnancy or child, or have a family member affected with the disease, are aware that they are at risk of transmitting an inherited disease. These couples, as well as those who have a positive serum or ultrasound screen, can be offered prenatal diagnosis. Chorionic villus sampling and amniocentesis have been the traditional methods of choice for prenatal diagnosis, and more recently, new molecular biology techniques have facilitated the development of noninvasive prenatal diagnosis tests that can screen fetal DNA in the maternal circulation.
Chorionic Villus Sampling (CVS)
CVS can be performed transcervically between 10 and 12 weeks of gestation, or from 12 weeks onwards by the transabdominal route. A sample of cells is removed from the placenta and used for diagnosis of chromosomal, metabolic and DNA analysis. The disadvantages of the procedure are that it cannot be used for neural tube and other congenital abnormalities, and some studies have suggested a risk of limb reduction deformities if it is performed too early or by inexperienced operators. There is also a 1–2% risk of miscarriage, which is a little higher than the risk after amniocentesis. In 1.5% of cases, the karyotype of the placenta is found to be different from that of the embryo (confined placental mosaicism).
Amniocentesis
Amniocentesis for prenatal diagnosis is usually performed in the second trimester, from 15 weeks onwards. Under ultrasound guidance, 15–20 mL of amniotic fluid is aspirated; this can be used for the diagnosis of chromosome abnormalities, measurement of specific substances, detection of inborn errors of metabolism such as Tay–Sachs disease, measurement of enzyme activity and diagnosis of neural tube defects. Its disadvantages include the potential of causing fetal loss (1%) and rarely there may be continued leakage of the amniotic fluid. The main limitation of this technique is that results are available only very late in the pregnancy (17–20 weeks), so that a termination must be induced during the second trimester.
Cell-Free Fetal DNA Screening (cfDNA)
Cell-free fetal DNA can be detected in maternal plasma as early as 7 weeks’ gestation, and this has been successfully used to provide noninvasive prenatal diagnosis (NIPD) for common aneuploidies, with low false positive rates. Cell-free DNA is extracted from a maternal blood sample, and real-time polymerase chain reaction (PCR) with fluorescently labeled specific probes for target genes is used to identify and quantify fetal-specific sequences present in low copy numbers. Accuracy depends on the condition under investigation: the test can accurately diagnose Rhesus D status, and fetal sex determination can identify X-linked disorders. Sensitivity and specificity are less for trisomy 21, 18 and 13 due to the influence of biological factors such as confined placental mosaicism (CPM). cfDNA screening is therefore currently considered as a screening test for aneuploidy, although accuracy is improving with developments in technology.
Fetal Blood Sampling, Cordocentesis or PUBS (Percutaneous Umbilical Blood Sampling)
These techniques are used less frequently than CVS or amniocentesis; samples can be taken from 18 weeks’ gestation to term. Fetal blood is taken from the cord or intrahepatic umbilical vein and used for fetal karyotyping (quick result), evaluation of fetal status (if an infection is thought to be present) and hematological abnormalities (Rh or immune hemolytic disease). The most common indication is karyotyping for single or multiple congenital abnormalities and mosaicism.
Diagnostic Testing for Prenatal Diagnosis
Tests used to establish a fetal diagnosis following CVS or amniocentesis include PCR and karyotyping. PCR analysis can detect single-gene defects and triplet repeat disorders and determine fetal gender. A karyotype is performed for any diagnosis which involves chromosome identification, i.e., in those patients carrying chromosome abnormalities or who are at risk of age-related aneuploidy. In some situations, fluorescent in-situ hybridization (FISH) or DNA microarray-based technology is used to complement the karyotype result.
Polymerase Chain Reaction
PCR is a molecular technique developed in the 1980s that is used to amplify a portion of DNA thousands of times. For prenatal diagnosis, PCR can be used to detect the normal or mutated gene by amplifying the region around the mutation. This is achieved using primers that have a sequence which is complementary to a region of the gene. Primers that will bind to either side of the mutation are selected, and copies of the DNA sequence in between (target gene) are generated via repeated cycles of heating and cooling:
-
1. Heating to 96ºC to denature the DNA and create separate strands
-
2. Cooling to 55º C to allow primers to bind to the DNA target (primer annealing)
-
3. Heating to 72ºC to allow DNA synthesis (primer extension).
This PCR cycle is repeated a number of times (25–35 times) to produce billions of copies of the DNA sequence for analysis. The reaction is made possible by using a thermostable polymerase enzyme that can withstand the high temperatures needed for denaturation: the first such enzyme to be used was Taq polymerase, isolated from a heat-tolerant bacterium (Thermus aquaticus) that lives in hot springs and hydrothermal vents. A similar enzyme, PfuDNA polymerase (from Pyrococcus furiosus), has superior thermostability and higher fidelity when copying DNA.
Once the DNA sequence of interest has been amplified, the PCR products are analyzed using a number of different techniques. The simplest method, which can be used to detect an insertion or deletion, is to separate the PCR products by polyacrylamide gel electrophoresis; other techniques include single-stranded conformational polymorphism (SSCP), amplification refractory mutation system (ARMS) and heteroduplex analysis, which can detect even just a single base change within a gene. The technology has further evolved over the past decade, with a range of more sophisticated DNA microarray molecular biology tools available (see Molecular Diagnosis).
Karyotyping
Karyotype analysis examines the chromosomes of a cell. For prenatal diagnosis, the sample obtained by CVS or amniocentesis is cultured to increase the number of cells, and mitotic inhibitors are used to arrest some of the cells in metaphase. Agents which elongate the metaphase chromosomes are also used. Slide preparations of the nuclei are treated with Giemsa stain, which results in a specific banding pattern for each chromosome. Using this method, missing or extra chromosomes, translocations, inversions, etc. can be identified. Occasionally the results of a karyotype may be inconclusive, and more advanced techniques are now used to help elucidate the diagnosis. Karyotyping has also been used to check the number of chromosomes in diagnosis of age-related aneuploidy.
Preimplantation Genetic Diagnosis
The technology required for preimplantation genetic diagnosis encompasses genetics, IVF, embryo biopsy and single-cell diagnosis. Since separate disciplines and techniques are required for embryo biopsy and diagnosis, PGD must be carried out by a genetics team in collaboration with an IVF team with access to molecular biology technology. The embryo biopsy technique should be performed by a trained embryologist, but the diagnosis must be performed by a molecular biology laboratory and confirmed by a genetics specialist.
History
The first children born as a result of preimplantation genetic diagnoses were reported in 1990 by the group of Alan Handyside in London, studying sex-linked diseases (Handyside et al., 1990). Robert Edwards had anticipated this development 22 years earlier, when he and Richard Gardner, his PhD student at the time, established a proof of principle by using blastocyst biopsy as a means of sexing rabbit embryos (Gardner & Edwards, 1968). By 2010 the number of children born in the world after screening embryos for genetic disease exceeded 10000; the HFEA in the UK currently licenses 419 genetic conditions for diagnosis by PGD.
The procedure for preimplantation genetic diagnosis involves:
-
1. Selection of a viable embryo
-
2. Collecting genetic material (embryo biopsy)
-
3. Preparation of the genetic material for analysis (DNA isolation and amplification)
-
4. Analysis of results and selection of the embryo for transfer to the mother.
Genetic analysis of embryos in fertility clinics currently has two different applications:
-
1. Preimplantation Genetic Diagnosis (PGD) for couples known to carry genetic disorders such as single-gene diseases or chromosomal translocations.
-
2. Preimplantation Genetic Selection (PGS or PGD-A), used to identify embryos with a normal karyotype.
The European Society for Human Reproduction and Embryology (ESHRE) PGD Consortium collects all European data on a regular basis, with published reports that summarize data pertaining to the cycles, from indication and methodology to cycle outcome. Their 14th Data Report (De Ryck et al., 2017) confirmed that during the period 2011–2013, preimplantation diagnosis for chromosome abnormalities, single-gene disorders and sexing for X-linked diseases was performed in 17 721 IVF cycles; PGS for aneuploidy was performed in 26 737 cycles during the same period, including 705 cycles for sex selection.
Embryo Biopsy
As outlined in Chapter 13, embryo biopsy is performed using micromanipulation equipment used for ICSI; all of the biopsy techniques involve two stages: zona drilling and aspiration (or herniation in the case of blastocyst biopsy). Cell biopsies can be taken from oocytes/embryos at three different stages:
-
1. Polar body biopsy in the unfertilized oocyte/zygote
-
2. Cleavage stage biopsy from the 6- to 8-cell embryo
-
3. Blastocyst stage biopsy.
Table 14.4 gives an overview of embryo biopsy methods; the techniques are discussed in detail in Chapter 13.
Day performed | Types of cells removed | Indications | Zona drilling | Cell removal | Limitations | |
---|---|---|---|---|---|---|
Polar body (PB) | First PB Day 0 Second PB Day 1 Or simultaneously on Day 1 | First and second polar bodies | PGS Monogenics carried by mother | Laser Mechanical Beveled pipette | Aspiration | Only maternal chromosomes/genes |
Cleavage stage | Day 3 | Blastomeres | PGS Monogenics Sexing Chromosome abnormalities | Laser Mechanical Acid Tyrode’s | Aspiration Displacement | Postzygotic mosaicism |
Blastocyst | Day 5 | Trophectoderm | PGS Monogenics Sexing Chromosome abnormalities | Laser Mechanical Acid Tyrode’s | Herniation | Postzygotic mosaicismSome embryos will arrest prior to biopsy |
Polar Body Biopsy
Biopsy of the first polar body was developed in order to overcome ethical objections to embryo biopsy, on the basis that the ‘ethical status’ of the unfertilized oocyte differs from that of the embryo. Some individuals opt for PGD in order to avoid termination of pregnancy, and performing the test on a preimplantation embryo may be just as objectionable as termination of pregnancy. Polar body biopsy was first used for the detection of CF; due to crossing-over events, the second polar body is also required in some situations. Polar body screening detects only maternal meiotic aneuploidies and will not identify errors that are due to paternal or postzygotic factors; biopsy of both polar bodies is recommended for PGD.
Cleavage Stage Biopsy
Biopsies performed at the four-cell stage may alter the ratio of inner cell mass to trophectoderm (TE) cells, which may be detrimental to embryo development. Therefore, biopsy at the six- to eight-cell stage, on Day 3 post-insemination, is preferred.
Several difficulties arise from cleavage stage embryo biopsy: the first is that human embryonic cells are very fragile and easily lyse. If this occurs during the biopsy procedure, the nucleus may be lost, and another cell will have to be removed. Compaction occurs between the eight-cell and morula stage, and during compaction the cells of the embryo can no longer be distinguished as they flatten out over each other to maximize intercellular contacts. If the biopsy is performed at this stage, it is very difficult to remove a blastomere, as it has established strong contact with adjacent blastomeres. Trying to remove a cell from a compacted embryo may also result in lysis of the cell. The thickness and dynamics of the zona pellucida also vary between patients and can lead to some problems during the biopsy procedure. In many cases, numerous sperm are associated with the zona pellucida, and therefore intracytoplasmic sperm injection (ICSI) should always be used with PCR techniques to reduce the risk of sperm contamination.
Studies of both cryopreserved/thawed embryos and those biopsied for PGD have shown that up to 50% of the embryo mass may be lost, and yet lead to a healthy live birth. Data from the ESHRE PGD consortium show that 97% of embryo biopsies were successful, with more than 90% of the embryos surviving. Pregnancies following embryo biopsy showed no significant developmental differences compared to controls. Deliveries, including infant birth weight and Apghar scores, were considered to be normal.
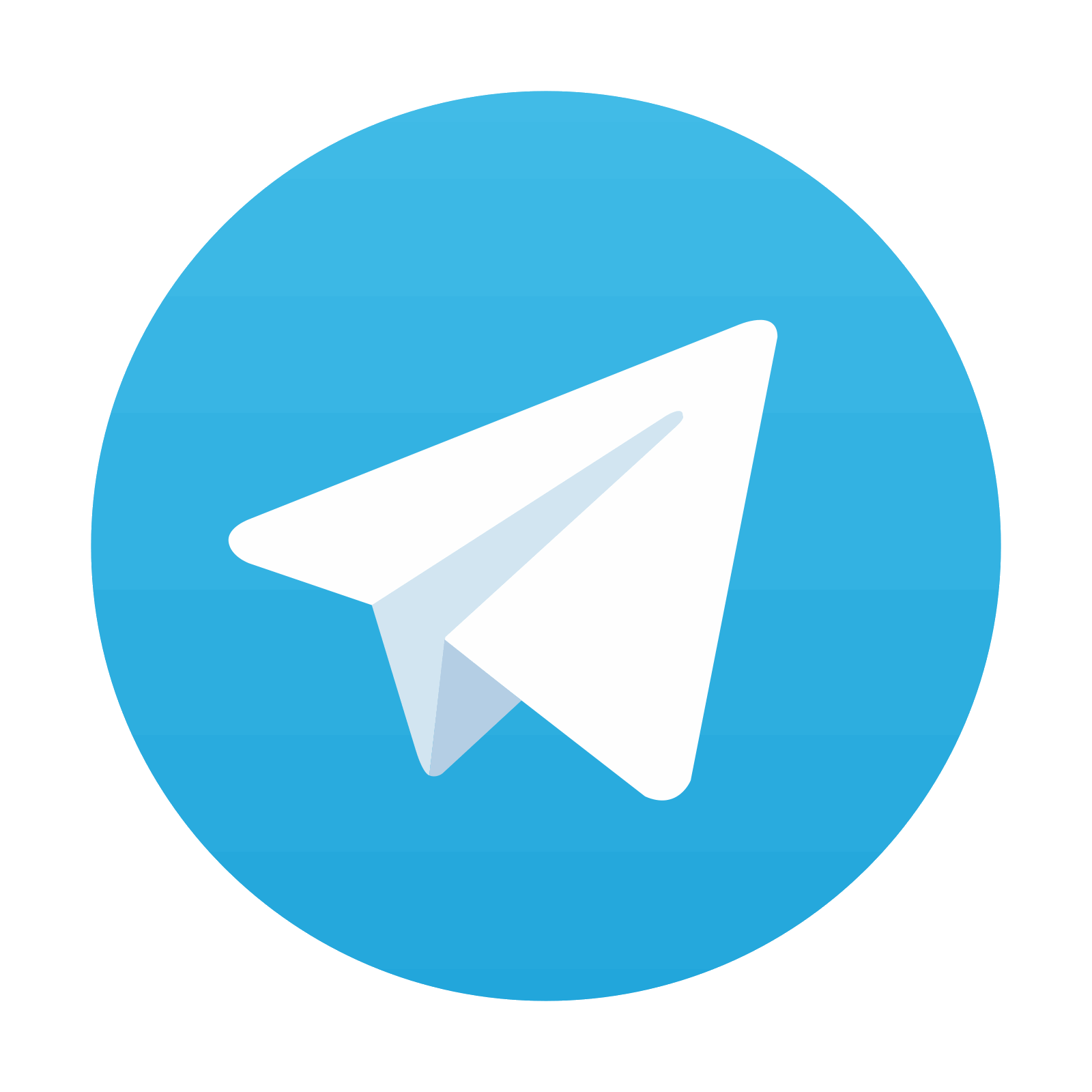
Stay updated, free articles. Join our Telegram channel
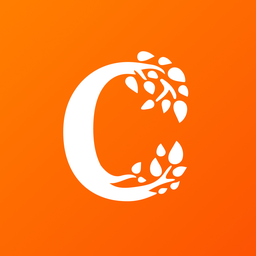
Full access? Get Clinical Tree
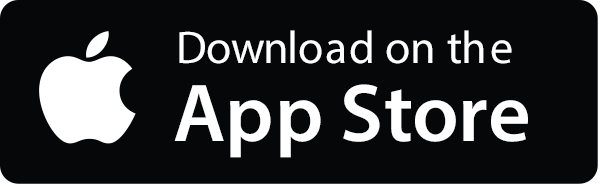
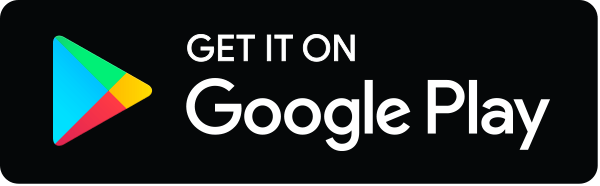