INTRODUCTION
Neuroimaging is a standard part of the evaluation of patients with focal epilepsy and any type of epilepsy in the setting of abnormal cognitive development. In this setting and with improvements in neuroimaging, malformations of cortical development are frequently recognized as the etiology of epilepsy in children.
The more aggressive pursuit of detailed neuroimaging in children with epilepsy is due in part to the more accepted role of focal resective epilepsy surgery as a treatment option in medically refractory focal epilepsy. Neuropathologic data from patients undergoing surgical resection of epileptogenic foci have shed further light on the prevalence and histopathologic properties of cerebral cortical malformations, even among some patients with normal magnetic resonance imaging (MRI). As such, the role of these malformations is proving to be substantial in the pathogenesis of refractory epilepsy in children.
We use the terms cerebral cortical malformation and malformation of cortical development interchangeably in this chapter to discuss malformations that represent known or presumed disruptions of brain development. Additionally, while cortical dysplasia can refer to the broad range of cerebral cortical malformations (1,2), we restrict our use of this term to discussions of focal cortical dysplasia (FCD) (3).
We begin by highlighting the role of malformations of cortical development in pediatric epilepsy. We then review what has become a well-established framework for the understanding and classification of these disorders, taking into account the central nervous system ontogeny and a growing understanding of the genes and pathways that regulate it. The role of germline and somatic mutations (pathogenic variants) in the understanding of brain malformations is expanding at a rapid rate and blurring the lines between the traditional categories of malformation types (4–7).
CEREBRAL CORTICAL MALFORMATIONS IN PEDIATRIC EPILEPSY
Type of Seizures and Epilepsy Associated With Cerebral Cortical Malformations
The majority of patients with malformations of cortical development and epilepsy have focal epilepsy. An individual with a cerebral cortical malformation may have seizures that appear generalized; such a patient most likely has secondarily generalized focal epilepsy, but generalized epilepsy is also possible. In these cases, under the most recently revised International League Against Epilepsy (ILAE) classification of seizures and epilepsy, the etiology would be specified as structural, due to the malformation of cortical development, though many of the malformations are genetic in origin (8).
Epidemiology of Cerebral Cortical Malformations in Pediatric Epilepsy
The role of cortical malformations has been widely recognized in cases of refractory focal epilepsy in children and adults, with malformations ranging from small heterotopia to large hemispheric malformations, such as hemimegalencephaly (3,9–13). Prevalence is higher at tertiary care centers and in refractory epilepsy patients than in population-based studies. A population-based study in Norway from 1999–2014 identified malformations of cortical development in only 31 (1.7%) of the 1,771 patients with active epilepsy of all ages (14). In an observational study of patients aged 15 years or older with focal epilepsy, only 70% of whom had any form of imaging performed, 3% of patients were found to have cerebral cortical malformations by MRI (15). However, 10% to 20% of patients seen in subspecialty epilepsy centers are estimated to have cerebral cortical malformations by some authors (11), and with improved imaging this number is likely even higher. In one series of adults with refractory epilepsy, 12% of patients had evidence of malformations of cortical development on MRI (16).
Epidemiologic studies performed in the era preceding the routine use of MRI do not reflect the role of malformations of cortical development in pediatric epilepsy, but there are more recent reports suggesting that their role is even greater in children than in adults with epilepsy, especially but not exclusively within epilepsy surgery series. There are additionally data to suggest that malformations of cortical development, cortical dysplasia in particular, is associated with early age of onset and shorter time to epilepsy surgery compared to hippocampal sclerosis with temporal lobe epilepsy and other etiologies (17,18). Kuzniecky et al reported that one quarter of a series of 44 children who underwent surgical resection for treatment of epilepsy demonstrated cortical malformations (19). Pasquier et al described 230 consecutive children and adults undergoing epilepsy surgery, the majority of whom had childhood-onset epilepsy; nearly one quarter had a cerebral cortical malformation, including FCD, tubers suggesting tuberous sclerosis complex (TSC), and dysembryoplastic neuroepithelial tumor (DNET) (20). Sinclair and colleagues described a series of 42 children undergoing temporal lobectomy, in whom four had pathologic evidence of FCD and four had tubers; additionally, of 13 patients who had temporal lobe tumors, eight fell into the spectrum of developmental malformations (seven gangliogliomas and one DNET) (21,22). Based on the ILAE survey of 20 pediatric epilepsy surgery centers in 2004, cortical dysplasia (including FCD and hemimegalencephaly) was the most frequent etiology in patients under 18 years (42% of 413 patients) (17,18). If undergoing epilepsy surgery in the first year of life, cortical dysplasia was identified in 67% of patients, compared to 25% of those aged 15 to 18 years (17,18). In a series of patients with infantile spasms collected by the Pediatric Epilepsy Research Consortium, 50/218 (23%) with MRI data had malformations of cortical development (23). Over a 10-year period at Boston Children’s Hospital from 2004 to 2014, 44% (124/280) of patients undergoing epilepsy surgery had malformations of cortical development visible on MRI (unpublished data).
In the subgroup of children undergoing epilepsy surgery for refractory infantile spasms, malformations of cortical development are a leading cause (24–26). Furthermore, there is a growing body of pathologic evidence from epilepsy surgery series demonstrating that many adults and children with intractable focal epilepsy without MRI abnormalities have malformations of cortical development that are currently appreciated only microscopically (11,27–31) or identified using 18FDG-PET and more recently 18FDG-PET/MRI coregistration (32,33).
To our knowledge, large population-based studies of children with epilepsy have yet to fully capture the role of cerebral cortical malformations. Nonetheless, the accruing evidence and our personal experience lead us to conclude that malformations of cortical development account for a substantial number of children with refractory focal epilepsy. They are also increasingly identified in children with epilepsy that is controlled or without epilepsy, both prenatally and postnatally.
CLINICAL PRESENTATION AND CLASSIFICATION OF CEREBRAL CORTICAL MALFORMATIONS ACCORDING TO STAGES OF CORTICAL DEVELOPMENT
The process of brain development, which follows a sequence that is under genetic regulation, provides a framework for understanding the variety of cerebral cortical malformations encountered in patients with pediatric epilepsy. The classification presented by Barkovich et al has evolved to include an ever-expanding list of genetic etiologies for many cerebral malformations (2,22,34). The importance of its emphasis on genetic causes cannot be overstated; even when epilepsy is not the presenting symptom of a cerebral malformation, clinicians must address important questions regarding possible genetic etiologies. Figure 6.1 provides a framework for the classification of malformations of brain development, first by disrupted stage of development and second by molecular pathways.
We begin this section with some general clinical observations regarding patients with epilepsy due to cerebral cortical malformations. We briefly review the normal sequence of cortical development before discussing representative examples from each major group according to the first stage of brain development known or believed to be disrupted.
General Clinical Observations
Apart from the minority of patients with cerebral cortical malformations who are asymptomatic, many patients with malformations of cortical development have an epileptic encephalopathy (8), manifest not only as epilepsy but also as varying degrees of developmental delay or intellectual disability as well as other neurologic problems. It is helpful to frame the individual patient’s neurologic problems, including the epilepsy, as stemming from the malformation of cortical development and to attribute the malformation itself, along with any associated nonneurologic sequelae, to the underlying genetic etiology whenever possible. The likelihood and typical age of onset of epilepsy vary among syndromes, and the most precise characterization of patients’ conditions will aid in the prognosis of their epilepsy as well as their neurodevelopmental outcome. We present a summary of key genes and copy number variants known to be associated with specific malformations of cortical development at the time of the writing of this chapter in Tables 6.1 to 6.3. Inheritance patterns include autosomal recessive and X-linked patterns, and noninherited mutations may be de novo germline or de novo somatic. Understanding the pattern of inheritance or lack thereof is critical in counseling families regarding recurrence risks.
FIGURE 6.1 Classification of malformations of brain development, evolution with updated molecular understanding adapted from Ref. (34). Barkovich AJ, Guerrini R, Kuzniecky RI, et al. A developmental and genetic classification for malformations of cortical development: update 2012. Brain. 2012;135(Pt 5):1348–1369. The Reelin-LIS1-tubulin pathway figure is reproduced with permission from Ref. (4). Guerrini R, Dobyns WB. Malformations of cortical development: clinical features and genetic causes. The Lancet. Neurology. 2014;13(7):710–726. Molecules in red are associated with migrational abnormalities.
HMEG-DMEG, hemimegalencephaly-dystrophic megalencephaly; MCAP, megalencephaly capillary malformation syndrome; MPPH, megalencephaly polymicrogyria polydactyly hydrocephalus syndrome.
The PI3K-AKT-MTOR pathway is reproduced with permission from Ref. (78). Mirzaa GM, Rivière JB, Dobyns WB. Megalencephaly syndromes and activating mutations in the PI3K-AKT pathway: mpph and MCAP. Am J Med Genet C Semin Med Genet. 2013;163C(2):122–130. Reprinted with permission from John Wiley & Sons.
The treatment of epilepsy due to malformations of cortical development is beyond the scope of this chapter, and general principles of treatment of epilepsy are covered in depth elsewhere in the text. Patients with refractory epilepsy due to localized and lateralized cerebral cortical malformations and seizure foci are often considered candidates for epilepsy surgery, and the outcome has been reported to be favorable in one-third to two-thirds of patients with brain malformations undergoing resective surgery for the treatment of seizures (157–159). Those patients with more diffuse and bilateral malformations may be considered candidates for palliative surgery in some circumstances.
TABLE 6.1
Classification by Stage of Cortical Development Disrupted and Presentations
Normal Cortical Development. The processes underlying normal cortical development in the human continue to be elucidated in increasing detail. The currently accepted paradigm for the formation of the human cortex, outlined elegantly by Sidman and Rakic in 1973 (160), includes the following sequence of events: (a) proliferation of progenitor cells in subventricular/ventricular zone, (b) neuronal migration along a radial glial scaffold, leading to the establishment of the six layers of the neocortex, and (c) further organization, including formation of gyri and sulci of the appropriate size and orientation, synaptic organization, and myelination. Figure 6.2 presents a schematic version of cortical development. This model for cortical development provides an excellent general framework and continues to be refined (161). Evidence that cortical neurons and the radial glia that guide their migration arise from a common neuronal-glial progenitor pool (162,163) suggests that there are other elements between the previously mentioned steps (a) and (b) that may be affected to produce malformations of cortical development, including the processes of cell fate determination and formation of the radial glial scaffold that must occur before migration can begin.
TABLE 6.2
TABLE 6.3
Disorders of Neuronal and Glial Proliferation. Disorders of neuronal and glial proliferation most likely result from abnormal proliferation of the undifferentiated progenitor of neurons and glia. These disorders reflect an imbalance between proliferation of progenitor cells and apoptosis, or programmed cell death. This category includes those conditions thought to be a result of relative underproduction of neurons and glia, or conversely those with a relative overproduction, as outlined in Figure 6.1 and Tables 6.1 and 6.2. Conditions resulting in the production of abnormal cell types are also included in this group, with both nonneoplastic and low-grade neoplastic subgroups (22,34). It is important to note that any perturbation at this early stage of development is likely to have downstream effects on the later stages, and it is therefore not surprising that cortical lamination and further organization also appear abnormal in this group of disorders.
FIGURE 6.2 The formation of the cerebral cortex in humans involves the proliferation of progenitor cells (light gray ovals) in the ventricular zone (VZ); establishment of a radial glial scaffold (vertical lines) following differentiation of some progenitors into glial cells (cell bodies shown as dark gray ovals) and others into neurons (patterned ovals); and neuronal migration along the radial glial scaffold. The panel on the left depicts the stage of approximately 16 to 18 weeks’ human gestation, by which time the layer I Cajal–Retzius neurons have migrated to the pial surface (represented by the top of the panel) followed by layer VI, layer V, and layer IV; representative neurons from layers III and II are shown migrating along radial glia. The panel on the right represents the adult six-layer cortex after neuronal migration is complete; note that the cortical plate has expanded with the addition of layers III and II. Not shown are the final stages of further cortical organization, including formation of gyri and sulci of the appropriate size and orientation, synaptic organization, and myelination.
MZ, marginal zone; SP, subplate neurons.
Net Decrease in Neurons and Glia—Microcephaly. Microcephaly can be the result of a number of etiologies including hypoxic–ischemic injury; exposure to viral pathogens in utero that result in an underproduction, excessive apoptosis, or abnormal destruction of neurons and glia during gestation; or genetic etiologies. It can be divided into congenital microcephaly and postnatal microcephaly, and the etiologies differ between these groups. In the case of congenital microcephaly, a number of genetic etiologies, primarily following an autosomal recessive form of inheritance, lead to disruption of the cell cycle and proliferation of neurons and glia. Mechanisms include centrosome and spindle microtubule defects (eg, ASPM), DNA damage response and repair defects (eg, PNKP), and origin recognition complex (ORC) defects. Seizures are a variably associated feature of the congenital microcephalies, including the most common cause of congenital microcephaly, mutations in ASPM for which epilepsy is reported in ~10% of individuals (164–166). Some of the other autosomal recessive genetic causes of congenital microcephaly such as PNKP and SLC25A22 are associated with severe early-onset epileptic encephalopathy nearly universally (41,42,167).
Neuroimaging of patients with primary congenital microcephaly typically show normal cortical structure or a simplified gyral pattern at times with reduced white matter volume and callosal anomalies (168,169), as shown in Figure 6.3. Some clinicians use the term microlissencephaly. Some of the causes of extreme congenital microcephaly have been more commonly associated with brain malformations. For example, homozygous or compound heterozygous mutations in WDR62 are associated with microcephaly and a variety of brain malformations such as schizencephaly, polymicrogyria, and pachygyria/lissencephaly spectrum (38–40). Similarly, homozygous loss-of-function mutations in NDE1 are associated with microlissencephaly, agenesis of the corpus callosum, cerebellar hypoplasia, and, rarely, hydranencephaly (44–46). Cortical malformations in combination with the microcephaly tend to increase the risk for epilepsy, although not all patients have epilepsy.
In patients with congenital microcephaly associated with pigmentary chorioretinopathy and dwarfism, mutations have been identified in the genes involved in centriole biogenesis including TUBGCP4, TUBGCP6, KIF11, and PLK4 (47–50).
FIGURE 6.3 Disorders of global brain size: microcephaly. FOXG1-related microcephaly at 35 months of age (A-D). A sagittal T1 image (A) demonstrates mild blunting of the rostrum of the corpus callosum. Note that the craniofacial ratio is normal despite marked postnatal microcephaly (z = −5). An overlay image (B) demonstrates minimal growth in head size between 9 months (inverse grayscale) and 35 months (grayscale) in age. Axial T2 and T1 weighted images (C, D) demonstrate mild gyral simplification and delayed myelination most noticeably in the frontal white matter. Profound microcephaly with simplified gyral pattern is imaged at 1 month of age (E, F). A sagittal T1 image (E) demonstrates a dramatically abnormal facial profile with the face disproportionately large relative to the brain. Axial T2 imaging (F) demonstrates profound simplification of the gyral pattern and prominent extra-axial spaces consistent with low volume; a small left subdural hemorrhage is present. Microlissencephaly in a newborn with pathogenic mutations in KATNB1 (Courtesy Brenda Barry, MS, CGC, and Christopher Walsh, MD, PhD). A sagittal T1 image depicts dramatic reduction of the craniofacial ratio (G), and coronal T2 imaging (H) demonstrates marked gyral simplification.
Postnatal microcephaly is associated with a number of well-established neurodevelopmental disorders commonly associated with epilepsy, including Rett syndrome and Angelman syndrome and their variants (51). Additional genes and syndromes associated with postnatal microcephaly and epileptic encephalopathy in at least some patients include CDKL5, FOXG1, CASK, QARS, PYCR2, Pitt–Hopkins syndrome (TCF4), Christianson syndrome (SLC9A6), Mowat–Wilson syndrome (ZEB2), and Warburg Micro syndrome (RAB3GAP1, RAB3GAP2) (Table 6.1) (51,52,56,64,66–68,70,79,170,171). Each of these disorders has characteristic clinical and, in some cases, radiologic features, which can help in the differential diagnosis. The epilepsy phenotype of each of these disorders is increasingly well defined (52,53,56,64,69–72,170–176).
The 16p11.2 locus is one of the epilepsy hotspots associated with increased risk for genetic generalized epilepsy (177–180). This region is an example of gene dosage effect on head size related to copy number variation (177). Patients with duplications in the 16p11.2 critical region have associated microcephaly, whereas patients with deletions have associated macrocephaly.
Net Increase in Neurons and Glia and Abnormal Proliferation—Megalencephalies, TSC, and FCD. Megalencephaly is seen in isolation or in association with syndromes associated with general dysregulation of growth in a number of tissues, including neurofibromatosis type 1, Cowden syndrome, and Sotos syndrome (87,181,182). Some individuals with these and other megalencephaly syndromes have epilepsy, but it is a less prominent feature compared to those with associated malformations as discussed later in this chapter (87,183,184). Examples of megalencephaly in Costello syndrome, Bannayan–Riley–Ruvalcaba syndrome, and megalencephaly capillary malformation (MCAP) syndrome are shown in Figure 6.4.
Abnormalities at the time of proliferation of neuronal-glial progenitor cells are thought to be responsible for the formation of cortical tubers in TSC, FCD with balloon cells, dysplastic megalencephaly, and hemimegalencephaly, all of which share many pathologic features (34,185–187).
Between 80% and 90% of individuals with TSC have epilepsy; seizures typically begin during childhood and often are refractory to medical treatment (188). In particular, children with TSC have a particular propensity for developing infantile spasms (189). Different aspects of epilepsy in children with TSC are covered in several other chapters in this volume.
FIGURE 6.4 Disorders of global brain size: macrocephaly. Macrocephaly due to Costello syndrome in a 3-year-old male (A, B). Sagittal T1 MRI (A) demonstrates marked frontal bossing and Chiari I malformation (arrow) with cervical syrinx (arrowhead) secondary to the large size of the brain. Axial T2 imaging (B) confirms there is a grossly normal size of the ventricles suggesting increased brain volume, megalencephaly, as the basis of the enlarged head circumference. An 8-year-old male with 10q23 deletion and Bannayan-Riley-Ruvalcaba syndrome (C, D) demonstrates frontal bossing and callosal dysgenesis on sagittal T1 imaging and a small cavernoma (arrow) in the right temporal lobe on SWI imaging (D). Clinically diagnosed megalencephaly capillary malformation syndrome with marked macrocephaly, polydactyly, and facial birthmark. A sagittal T2 image (E) demonstrates marked frontal bossing and a Chiari I malformation (arrow), in this instance accompanied by ventriculomegaly (arrowheads). The lateral sagittal T2 images demonstrate diffuse polymicrogyria (F).
Hemimegalencephaly is a large, hemispheric malformation with varying degrees of macroscopically disrupted architecture. There are at times microscopic or milder macroscopic abnormalities in the contralateral hemisphere. There are cases in which one lobe or a portion of a hemisphere appears affected, likely on a spectrum with the FCDs. Some authors use the broad term dysplastic megalencephaly to refer to a spectrum that includes these more focal cases but also those with bilateral involvement. Dysplastic megalencephaly has similar imaging and pathologic features to FCD type II (3,34). Hemimegalencephaly can occur as an isolated entity or as part of a syndrome with somatic components, such as linear sebaceous syndrome, hypomelanosis of Ito, Proteus syndrome, and rarely but not surprisingly other neurocutaneous syndromes such as TSC and neurofibromatosis type 1 (92,190,191).
A distinct spectrum of overgrowth disorders characterized by megalencephaly or hemimegalencephaly along with a spectrum of other clinical features such as vascular anomalies are linked to activating mutations of the PI3-kinase-AKT pathway, often somatic. They include both germline (PIK3R2, PIK3CA, DEPDC5, PTEN) and somatic (AKT3, PIK3CA, CCND2, mTOR, TSC2, DEPDC5) mutations with some phenotypic specificity but also overlap (5,77–79,83–85). In many cases, activating missense mutation variants are identified, but duplications including one of the genes is another identified mechanism (84). Genes in this pathway are linked to the overlapping syndromes, megalencephaly polymicrogyria polydactyly hydrocephalus (MPPH, germline activating mutation variants in PIK3R2 or AKT3) syndrome and MCAP. Additional overlapping syndromes with more systemic features include congenital lipomatous overgrowth, vascular malformations, epidermal nevi, and skeletal/spinal abnormalities (CLOVES syndrome) (caused by PIK3CA heterozygous activating somatic mutations), Proteus syndrome (caused by AKT1 heterozygous activating germline mutations), and polyhydramnios, megalencephaly, and symptomatic epilepsy syndrome (PMSE or pretzel syndrome, caused by homozygous mutations in LYK5/STRADA) (192–194). MPPH is characterized predominantly by megalencephaly with or without associated features such as perisylvian predominant polymicrogyria, ventriculomegaly or hydrocephalus, Chiari malformation, thick corpus callosum, or postaxial polydactyly (78). MCAP syndrome is differentiated by its association with cutaneous vascular (capillary) malformations and variably associated features including asymmetric somatic overgrowth, connective tissue dysplasia, cutaneous syndactyly, polysyndactyly, and structural cardiac abnormalities or arrhythmias (78). Examples of imaging for this spectrum of disorders, including dysplastic megalencephaly and, on the other end of the spectrum, focal cortical dysplasia type IIb are shown in Figure 6.5. Focal cortical dysplasias type IIa and IIb are included in this group of disorders with abnormal proliferation but discussed together with other types of cortical dysplasias at the end of this section of the chapter.
Linear sebaceous nevus syndrome is related to somatic activating mutations in the RAS-MAPK pathway, specifically HRAS and KRAS (93). This disorder is in some cases associated with hemimegalencephaly as previously noted. This is a parallel growth pathway to the PIK3 kinase pathway discussed earlier, and is shown in Figure 6.1.
Epilepsy inevitably occurs in patients with hemimegalencephaly, and hemispherectomy is often a necessary component of seizure management (168). Many patients with hemimegalencephaly present in the neonatal or early infantile period with refractory epilepsy. They may be frequently hospitalized until hemispherectomy.
FIGURE 6.5 Dysplastic megalencephaly and focal cortical dysplasia type IIb.
–Right frontal focal cortical dysplasia type IIb in a 6-year-old with intractable seizures and F4 spikes on EEG. Coronal FLAIR (A) demonstrates signal increase in the right frontal and insular cortex (dashed circle) with a tapering extension toward the ventricular margin, the so-called “transmantle sign.” Sagittal T1 weighted images through the right frontal abnormality (B) and the corresponding location in the left frontal lobe (C) depict blurring of the gray-white matter differentiation only on the affected right side (B, dashed circle). Axial FLAIR (D) images redemonstrate the transmantle sign in another plane.
–Right hemimegalencephaly with somatic E17K AKT3 mutation in affected tissue (E,F). Axial and coronal T2 weighted images (E,F) demonstrate enlargement of the right frontal and temporal lobes with thickened, polymicrogyric cortex and a background of abnormal T2 shortening for a patient with an age of 3 months.
–A 5-month-old infant with more extensive right hemimegalencephaly. The axial T1 weighted image (G) demonstrates a broadened gyri with thickened cortex and the appearance of accelerated myelination in the larger right hemisphere. There is associated enlargement of the occipital horn and deviation of the right occipital horn to the left. Axial and coronal T2 weighted images through the posterior fossa (H,I) demonstrate the right cerebellar hemisphere and right globe are also enlarged.
–Intractable seizures in a 6-month-old with right hemibody hemihypertrophy and verrucous plaques consistent with linear sebaceous nevus syndrome. Axial (J) and coronal (K) T2 weighted images demonstrate enlargement of the right temporal lobe compared to the left side, accompanied by some patchy areas of dysmyelination (arrowhead, K). Sagittal T2 images (L) demonstrate subtle nodularity consistent with polymicrogyria in the right temporal lobe (dashed oval) not present on the contralateral side (M). Note the right temporoparietal scalp lesion (asterisk) partially visualized in image (L).
–A 3-year-old with megalencephaly, right-sided overgrowth, syndactyly, and facial capillary malformation subsequently found to have a PIK3CA mutation. Sagittal T1 imaging (N) demonstrates frontal bossing and a Chiari I malformation (arrow) reflecting megalencephaly. There is also marked thickening of the genu of the corpus callosum (dashed arrow). Left and right parasagittal T1 images (O, P) demonstrate perisylvian polymicrogyria extending over the frontal lobes (dashed circles). Axial T2 weighted imaging (Q) demonstrates prominent perivascular spaces in the enlarged corpus callosum (arrowheads) and the perisylvian polymicrogyria (dashed circles).
Abnormal Neoplastic Proliferation—Developmental Tumors. Glioneuronal tumors including dysembryoplastic neuroepithelial tumor (DNET) and ganglioglioma as well as gangliocytoma are developmental tumors with abnormal proliferation of progenitor cells that are associated with epilepsy. They have been reported in a series of children undergoing epilepsy surgery with varying frequency (21,34). There is evidence of over-activation in the mTOR signaling pathway in glioneuronal tumors, as in focal cortical dysplasia IIb, hemimegalencephaly, and tubers in tuberous sclerosis (195). Additionally, BRAF V600E mutations are identified in up to 50% of gangliogliomas, desmoplastic infantile gangliogioma, and DNETs (195,196). BRAF is linked to the RAF-MEK-ERK-MAP pathway, upstream of the mTOR pathway. These related pathways are the likely link between these epileptogenic developmental tumors and other malformations of cortical development (195).
Disorders of Neuronal Migration and Cortical Lamination. Disorders of neuronal migration include a group of disorders with macroscopic disruptions in cortical architecture, resulting in either absent or profoundly abnormal cortical lamination. Classic examples in this category include lissencephaly as well as the X-linked conditions of double cortex syndrome and periventricular heterotopia, discussed in detail in the following text.
Theoretically, a disruption at the earlier stage of proliferation could establish an abnormal substrate of neurons and glia leading to abnormalities in migration and lamination. For the disorders in this group, it is helpful to conceptualize them as those with a dominant defect in migration with possible origin from an earlier defect or with defects at both the proliferation and migration stages. Indeed, molecular evidence from studies of LIS1, a gene classically associated with neuronal migration, demonstrates a role for the gene at the stage of progenitor proliferation in the developing mammalian brain (197). That these disorders, primarily attributed to defects in radial migration, are also associated with abnormalities in gyral folding emphasizes that defects in early stages of development will result in disruption of later stages. As additional genetic causes of lissencephaly and related malformations are identified, it is clear that many of the molecular abnormalities lead to disruption at multiple stages of development and can be associated with a spectrum of related malformations. We attempt to classify them by their predominant associated feature, realizing that there is considerable overlap.
Abnormal Radial Migration—Classical Lissencephaly and Subcortical Band Heterotopia. Classic examples of these malformations of cortical development include the conditions of lissencephaly and double cortex syndrome, representing abnormal radial migration of neurons. Lissencephaly, meaning “smooth brain,” affects the entire cortex but can do so variably depending on the underlying genetic etiology. It is helpful to think of classical, or type I, lissencephaly as a spectrum from complete lissencephaly (agyria) to partial lissencephaly (pachygyria) with either an anterior–posterior or posterior–anterior gradient of severity (198). Epilepsy and severe intellectual disability are nearly universal features of classical lissencephaly. Epilepsy is early onset in the majority, and often includes infantile spasms. On the other hand, females with double cortex syndrome, or bilateral subcortical band heterotopia, typically have mild to moderate intellectual disability and epilepsy that is generally less severe than in individuals with lissencephaly (199,200).
Classical lissencephaly occurs in both males and females. Deletions or mutations in the LIS1 gene (alternate gene name PAFAH1B1) are the most common cause of lissencephaly, accounting for ~65% of the classical isolated lissencephaly sequence (4,127,201). The posterior-predominant agyria accompanied by anterior pachygyria is typical of LIS1-associated lissencephaly. When there is a deletion of LIS1 and other genes in the 17p13.3 region, the affected individuals have not only lissencephaly and its consequences but also the dysmorphic features that comprise the Miller–Dieker syndrome (128). Figure 6.6A,B present the MRI from a patient with Miller–Dieker syndrome. Somatic mosaicism for LIS1 mutations can also cause subcortical band heterotopia (127,202).
Classical lissencephaly or anterior predominant pachygyria in males occurs as a result of mutations in the DCX gene (128,131,132,203). Females with DCX mutations typically display double cortex syndrome, and their male offspring have a 50% risk of inheriting a mutated form of DCX and presenting with classical lissencephaly. Mutations include single amino acid substitutions and protein-truncating mutations. Somatic mutations in patients and germline mosaicism in parents have been observed (5,133,204). Figure 6.7 shows a range of patients with mutations in the DCX gene, including classical lissencephaly in a boy, pachygyria in a boy, and double cortex in a girl.
Mutations in the α–tubulin gene, TUBA1A, are associated with a spectrum of malformations, including lissencephaly, but also in some cases polymicrogyria with overmigration into the meningeal space. In the case of mutations at the amino acid position Arg402 in the TUBA1A gene, the imaging findings are indistinguishable from the classical lissencephaly seen with LIS1 mutations or deletions, at least in some cases (136). With other mutations in TUBA1A, there is also lissencephaly with a posterior predominance as well as dysmorphic ventriculomegaly, dysmorphic basal ganglia, cerebellar hypoplasia, and absent or hypoplastic corpus callosum. The basal ganglia abnormalities can be a clue to this particular disorder. An example of a patient with posterior predominant pachygyria with a TUBA1A mutation is shown in Figure 6.6C,D and compared to LIS1 associated posterior predominant pachygyria. Mutations in TUBA1A explain approximately 30% of cases of lissencephaly with cerebellar hypoplasia and approximately 1% of cases of classical lissencephaly (127,136). Subsequently, a spectrum of pachygyria/lissencephaly has been identified in association with mutations in related genes involved in Reelin-mediated signal pathways for neuronal migration (Figure 6.1) including TUBA1A, TUBB2B, DYNC1H1, KIF2A, and TUBG1 (4,5,108–110,136,137).
Focal abnormalities of migration, such as focal subcortical heterotopia, are also seen in association with refractory epilepsy (205). Two examples of focal heterotopias are shown in Figure 6.8. Genetic causes of isolated subcortical heterotopia have yet to be identified. The focal nature of these lesions makes them attractive candidates for epilepsy surgery, though long-term outcome data are not available.
Abnormal Radial Overmigration—Cobblestone Lissencephaly. Type II lissencephaly, or cobblestone malformation, superficially resembles classical (type I) lissencephaly because of the thickened cortex with simplified gyral pattern. However, cobblestone malformations are generally distinguishable from classical lissencephaly with modern high-quality MRI due to the bumpy surface of the cortex, islands of gray matter deep to the cortex, and areas of dysmyelination. This difference in radiologic appearance reflects a different pathophysiologic mechanism of overmigration of neurons beyond the pial surface. While overmigration into the leptomeninges is well established in cobblestone lissencephalies, it is reported to be seen on pathology in polymicrogyria with a variety of etiologies genetic and acquired (206). There is a strong clinical association between cobblestone lissencephaly and congenital muscular dystrophy in conditions such as Walker–Warburg syndrome, muscle eye brain disease, and Fukuyama muscular dystrophy (205,207–209). These are autosomal recessive disorders associated with mutations in genes associated with o-linked glycosylation, including POMT1, POMT2, POMGNT1, FKTN (FCMD), FKRP, LARGE, and ISPD (127,143–145). An example of cobblestone lissencephaly in a patient with pathogenic ISPD mutations is shown in Figure 6.9A–D. While these are the typical disorders associated with cobblestone lissencephaly, the tubulinopathies have similar features with overmigration of neurons into the meningeal space. Mutations in GPR56, believed to be the cause of fronto-parietal-polymicrogyria based on imaging features, has been reclassified as a cobblesone malformation due to the pathologic pattern of overmigration as well. Typical imaging in a patient with pathogenic GRP56 mutations is shown in Figure 6.9E–G. Epilepsy is often an early component of the cobblestone lissencephaly syndromes, which are associated with other MRI abnormalities, such as varying degrees of intellectual disability, hypotonia, and weakness (Table 6.3).
FIGURE 6.6 Severe, posterior predominant pachygyria in a 4-year-old with Miller–Dieker syndrome (A, B). Sagittal T1 (A) brain MRI demonstrates mild callosal hypoplasia and subjectively reduced brainstem volume. Axial T2 (B) demonstrates markedly thickened cortex and classic lissencephaly apart from some rudimentary gyri present anteriorly. Of note, the basal ganglia are normally cleaved.
–Newborn with TUBA1A mutation and severe posterior predominant pachygyria (C). Axial T2 (C) demonstrates lissencephaly apart from a few rudimentary gyri anteriorly. Unlike the Miller–Dieker case, the basal ganglia are fused (asterisk, C), which is common for tubulinopathies. A sagittal T1 weighted image (D) demonstrates cerebellar hypoplasia.
FIGURE 6.7 These axial images show a range of MRI abnormalities in patients with epilepsy and mutations in the DCX gene range from (A) lissencephaly in a boy, (B) pachygyria in a boy, and (C) double cortex in a girl. These cases illustrate global disruptions of neuronal migration.
Abnormal Radial Migration—Periventricular Heterotopia. X-linked bilateral periventricular heterotopia (PH) provides another example of abnormal radial neuronal migration, in this case with the appearance of arrested or minimal migration of a subset of neurons from the subventricular zone during cortical development. Figure 6.10 is an MRI from a female patient with this form of malformation due to a mutation in the FLNA gene on the X chromosome, the typical genetic abnormality associated with X-linked bilateral PH (146–149). Females with bilateral PH usually have epilepsy, with age of onset in their teens or twenties and milder severity than patients with the other disorders of migration; cognition is often normal, though specific defects in reading ability have been identified (210). Less commonly, this condition can occur in males with a similar overall phenotype or with a more severe neurodevelopmental and epilepsy phenotype including West syndrome in some (147,150,152). Not all females with bilateral PH harbor mutations in FLNA (147); another gene for which mutations have been associated with PH is ARFGEF2, described in autosomal recessive pedigrees with PH and microcephaly (153) and classified by Barkovich et al as a disorder of neuronal proliferation (34). Additionally, copy number variation in several chromosomal locations has been reported in association with periventricular nodular heterotopia including 5p15, 5q14.3, 6p25, 6q27 (terminal 6q deletion syndrome), and 7q11.23 (Williams syndrome) (4). We have additionally observed PVNH and associated epilepsy in a patient with mosaic inverted duplication on chromosome 13q31.2–q34. Of these, the terminal 6q deletion syndrome is the only one with an associated candidate gene, C6orf70 (125).
FIGURE 6.8 Other gray matter heterotopias.
–A 7-year-old male with a history of fenestrated interhemispheric cyst and shunted hydrocephalus. Axial T2 (A) and coronal T2 (B) demonstrates a mass-like conglomeration of gray matter with dilated perivascular spaces (arrow), consistent with subcortical gray matter heterotopia.
–A 5-year-old male imaged for hemophagocytic lymphohistiocytosis found to have left frontal transmantle gray matter heterotopia. Axial T2 (C) and sagittal oblique T1 (D) images show a centrifugal focus of gray matter (arrow) between the left frontal horn and the left frontal cortex.
FIGURE 6.9 Cobblestone malformation.
A 3-month-old infant with Walker–Warburg syndrome secondary to pathogenic ISPD mutations and congenital hydrocephalus (A-D). Axial T2 imaging demonstrates a thick and unfolded cerebral cortex with small gray matter rests within the subcortical white matter (inset, A). Dependent pus and a right frontal drainage catheter are present from ventriculitis, which complicated shunt catheter placement. A coronal T2 image (B) demonstrates tiny cerebellar cysts (largest left cyst marked with arrow). Sagittal FIESTA (C) demonstrates a marked cerebellar hypoplasia (arrow) and kinked appearance of the brainstem (asterisk). Axial T2 weighted imaging (D) demonstrates a persistent hyperplastic primary vitreous on the left side (arrow). A 2.5-year-old patient with developmental delay and frontal predominant cobblestone malformation was subsequently found to have compound heterozygous mutations in GPR56 (E-G). Sagittal T1 (E), axial T2 (F), and coronal T2 (G) weighted images demonstrate the frontal predominant cortical thickening and increased gyral frequency (cobblestone malformation) as well as subcortical white matter signal increase (dysmyelination) typical of GPR56 mutations. While abnormal gyration and cortical thickening are reminiscent of polymicrogyria, the pathology series is indicative of cobblestone malformation.
FIGURE 6.10 A 15-year-old female with hyperextensibility, seizures, and brain findings typical of FLNA periventricular nodular gray matter heterotopia (A-D). Axial T2 (A), axial T1 (B), and coronal T1 (C) images demonstrate mass-like conglomerates of heterotopic gray matter coating the length of the lateral ventricles. The sagittal T1 image (D) demonstrates co-occurrence of a mega cisterna magna (asterisk).
Abnormal Radial and Tangential Neuronal Migration—XLAG. The last example in this section represents a disorder in which both radial and tangential migration of neurons is affected. Tangential migration involves GABAergic interneurons from the median ganglionic eminence. X-linked lissencephaly with abnormal genitalia (XLAG) is the most severe phenotype described in association with mutations in the ARX gene (211). The lissencephaly is posterior-predominant, similar to classical lissencephaly seen with LIS1 mutations, and may be accompanied by agenesis of the corpus callosum and basal ganglia abnormalities (212). Also notable are ARX mutations in nonsyndromic boys with infantile spasms without lissencephaly (134) but presumably with disrupted migration at a microscopic level. Similarly, Ohtahara syndrome is reported in a subset of males with pathogenic ARX mutations, at times with dysgenesis of the corpus callosum, atrophy, and/or white matter abnormalities but without lissencephaly (213–216). Females with ARX mutations can have agenesis of the corpus callosum in some cases, and neurocognitive and psychiatric symptoms in others (213,217).
Disorders of Later Cortical Organization. Disorders of later cortical organization chiefly include polymicrogyria (PMG) and schizencephaly. FCD without balloon cells and lesions that are evident only by microscopic analysis are included in this category as well (FCDIa-c and IIa-d). All of these conditions are associated with epilepsy.
PMG can be created experimentally by freeze lesions in a rodent cortex (218), suggesting that local disruptions of either genes or the environment during human brain development could produce similar focal lesions in humans. PMG has also been associated with diverse etiologies including in utero exposure to cytomegalovirus, metabolic disorder such as disorders of peroxisome function and fumarase deficiency, and a variety of genetic etiologies (104,117,120,121,187,219,220). Additionally, it can be found adjacent to areas of vascular injury or paramedian interhemispheric cysts that occur during development, and can be a secondary finding in Sturge–Weber syndrome along with FCD within the affected hemisphere (221,222). As the genetic and pathologic understanding of the polymicrogyria syndromes advance, it has become clear that it is a migrational abnormality found in a heterogeneous group of disorders and not a single entity. The radiologic appearance is similar, however, with a few distinct patterns helping to subgroup the polymicrogyria types. Bilateral perisylvian polymicrogyria is the most common pattern. Examples of polymicrogyria of infectious or genetic origin are shown in Figure 6.11. Epilepsy has been a defining feature of the bilateral PMG syndromes, occurring in the vast majority of cases, and these conditions bear further discussion.
The bilateral perisylvian PMG (BPP) syndrome consists of epilepsy and a characteristic oromotor apraxia; epilepsy occurs in nearly 90% of affected individuals (223). Perisylvian polymicrogyria is associated with mutations in the “tubulinopathy” group including DYNC1H1, KIF5C, TUBA1A, TUBA8, TUBB2B, TUBB3, and TUBB5 (221,222). Among these, TUBA1A is the best established and likely the most commonly identified. As noted, mutations in this gene cause a spectrum of migrational abnormalities from lissencephaly to polymicrogyria. BPP has also been described in association with chromosomal deletions including most notably 22q11.2, 1p36, and 6q26–27, among others less frequently (122,224,225). When in combination with megalencephaly, the PI3 kinase-AKT pathway disorders (MCAP/MPPH) are most commonly associated as previously discussed. Thus, BPP is a genetically heterogeneous syndrome.
The bilateral fronto-parietal PMG (BFPP) syndrome—which includes epilepsy, intellectual disability, dysconjugate gaze, and pyramidal signs—has been associated with mutations in the gene GPR56 (105,106,107,226,227). This disorder has been reclassified as a cobblestone malformation based on histopathologic data, but it is still considered in the differential diagnosis of polymicrogyria because the abnormal gyral folding in this disorder (as well as for at least some TUBB2B cases) cannot always be readily distinguished from generic polymicrogyria at MRI (196,228). Suggestive imaging findings include patchy to confluent white matter T2 signal changes, brainstem and cerebellar hypoplasia, and cerebellar cysts (104,107). Interestingly, in a series of 13 patients with a form of bilateral PMG restricted to the frontal regions, only five had epilepsy (229). Mutations in the gene OCLN1 also cause fronto-parietal PMG with band-like subcortical calcifications and progressive atrophy (116).
Causes of generalized polymicrogyria include metabolic disorders such as fumarase deficiency and the peroxisomal disorders (104,117,118,120,121). Zellweger syndrome and related peroxisomal disorders are associated with generalized PMG in association with a leukoencepholopathy, atrophy, and germinolytic cysts in the caudothalamic groove (120,121). Cytomegalovirus is associated with perisylvian predominant or at times generalized polymicrogyria along with other imaging features such as white matter changes, periventricular cysts, and/or calcifications and clinical features such as sensorineural hearing loss and microcephaly (199,220,230).
Schizencephaly is a cerebral malformation consisting of one or more clefts that span the depth of the cortex, from the lateral ventricle to the pial surface, and that are lined by gray matter (231). The gray matter lining the clefts is polymicrogyric in appearance and the migrational abnormality can extend to the cortex and into the ventricles, as demonstrated in Figure 6.11M,N. Similar to polymicrogyria, schizencephaly can occur unilaterally or bilaterally, and can result from a variety of viral, vascular, or less often, genetic abnormalities (231–233). While genetic etiologies are typically not identified in the case of schizencephaly, mutations in COL4A1 have been identified in some cases (234). Mutations in this gene additionally cause a broader range of phenotypes including fetal hemorrhage and porencephaly (234–236). Schizencephaly is one of the more commonly associated cortical malformations seen in association with septo-optic dysplasia, as in the example in Figure 6.11M,N (237,238). About half of the individuals affected with schizencephaly are reported to have epilepsy, and as yet there is no clear correlation between the type of schizencephaly and the occurrence or severity of epilepsy in these patients (239). Patients with homozygous or compound heterozygous WDR62 mutations have congenital microcephaly, often with associated malformations including schizencephaly, polymicrogyria, or lissencephaly (38–40).
FIGURE 6.11 Spectrum of imaging manifestations seen in polymicrogyria (dashed circle indicates areas of polymicrogyria). Bilateral perisylvian PMG on axial T1 imaging of an adult male with bilateral retinoblastoma (A); asymmetric perisylvian PMG on axial T1 MRI from a 3-year-old with seizures (B); axial T2 image demonstrates asymmetric frontal predominant PMG in a 3-month-old with right-sided convulsions and rightward eye deviation (C); a 12-year-old female with congenital CMV demonstrates microcephaly and cerebellar hypoplasia (arrow) on sagittal T1 imaging (D), diffuse PMG and periventricular calcification on axial T1 imaging (E arrows), and white matter gliosis on coronal T2 weighted images (F, arrows); axial and coronal T2 images (G, H) demonstrate asymmetric perisylvian PMG in a newborn with 22q11 deletion syndrome; a 3-year-old boy with seizures and bilateral perisylvian PMG and basal ganglia fusion (asterisks) on an axial T1 image (I) was subsequently found to have a pathogenic TUBA1A mutation; a 4-month-old with pathogenic TUBA1A mutation and microcephaly was found to have fused basal ganglia (asterisk, J) and perisylvian PMG on axial/coronal T2 weighted images (J, K); a 17-year-old with seizure and left parietal transmantle gray matter heterotopia (asterisk, L) and overlying left temporo-parietal PMG as seen on a coronal T1 image; MRI of a 6-year-old girl with septo-optic dysplasia demonstrates deficiency of the septum pellucidum (asterisk) and right parietal schizencephaly on coronal T2 weighted imaging (M), extensive PMG along the schizencephalic defect on a right parasagittal T1 image (N), and contralateral PMG in a left parasagittal T1 image; MRI from a 14-year-old girl with endocrine deficiency and visual loss consistent with septo-optic dysplasia demonstrates absence of the septum pellucidum (asterisk, P), hypoplastic optic apparatus (arrow, P), and hypoplastic right olfactory apparatus (arrow, Q), as well as right inferior frontal PMG (R).
FOCAL CORTICAL DYSPLASIAS
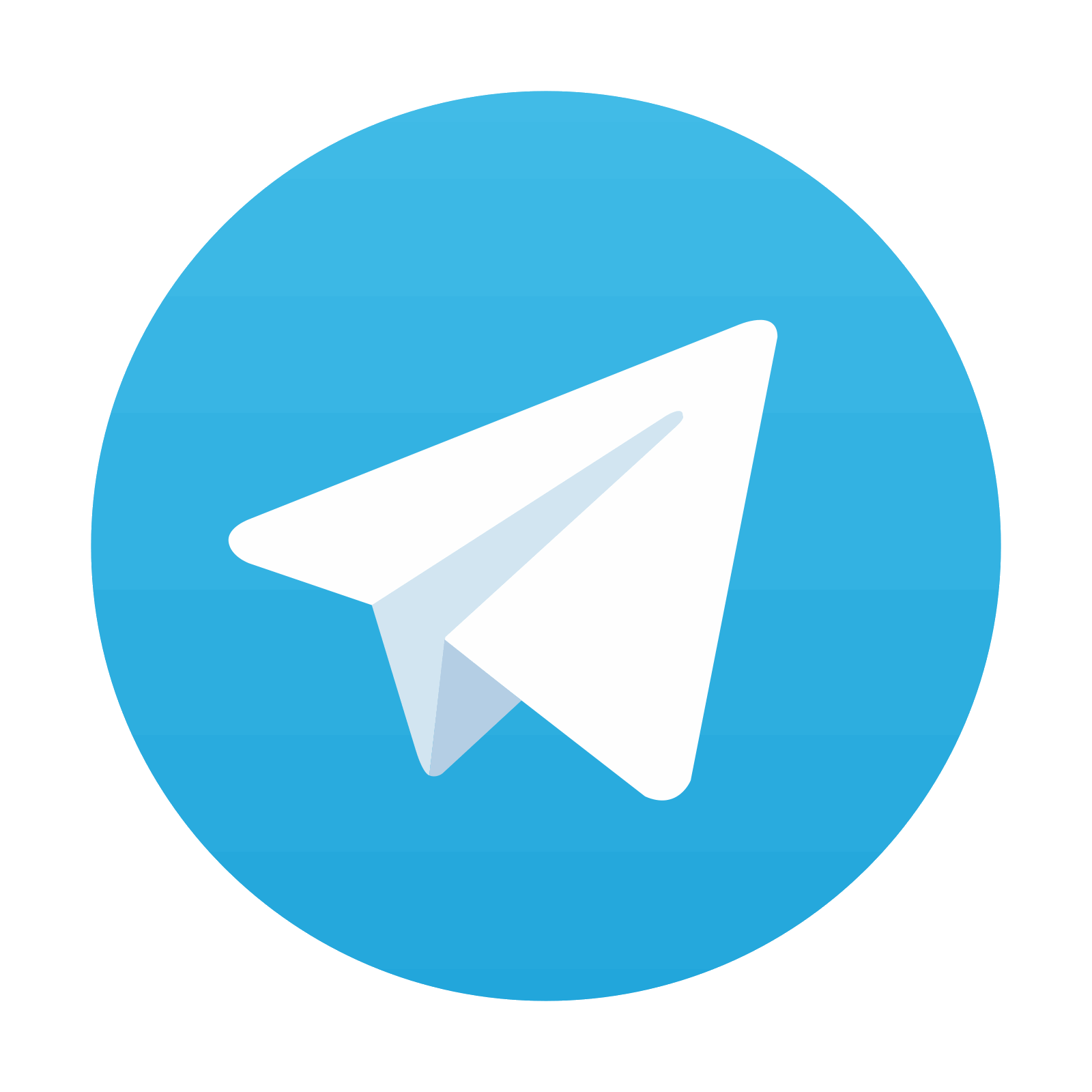
Stay updated, free articles. Join our Telegram channel
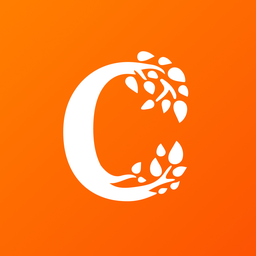
Full access? Get Clinical Tree
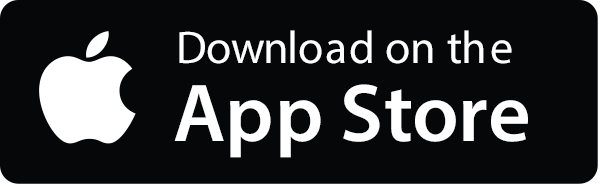
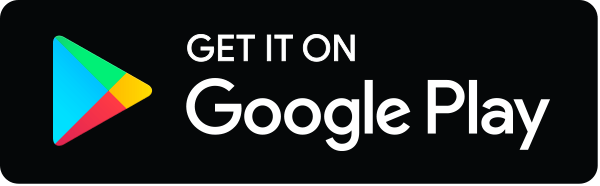