- •
The postnatal lung contains populations of stem cells with the capacity to reconstitute the lung following injury.
- •
Prematurity and its antecedent factors may alter lung stem cell programming leading to dysfunctional repair.
- •
Preterm infants in whom bronchopulmonary dysplasia develops exhibit decreased and/or dysfunctional stem cells.
- •
Preclinical studies provide robust evidence that stem cells reduce lung injury and improve survival in experimental models of bronchopulmonary dysplasia via paracrine-mediated mechanisms.
- •
Mesenchymal stem cells are an attractive population for lung repair as they are immunoprivileged, easily isolated, and have pleiotropic therapeutic effects.
- •
Early clinical evidence demonstrates the safety and feasibility of mesenchymal stem cell therapy for bronchopulmonary dysplasia. However, barriers for implementation, including optimal dosing, patient, timing, and culture techniques, need to be overcome for successful translation.
The lungs are remarkably complex organs with more than 40 different cell types uniquely organized to facilitate gas transport and exchange. This intrinsic complexity along with low cell turnover has led to challenges in understanding lung stem cell biology. Despite this, real progress has been made in the past decade and recent reports indicate that following injury, the damaged lung epithelium has the capacity to repair itself by a population of resident lung stem cells, with possibly minor contribution of bone marrow (BM)-derived stem cells. More data are also accumulating on the complex niches within which stem cells reside and the molecular mechanisms that regulate stem cell self-renewal and differentiation, and their impact on disease pathogenesis. Evidence is also mounting that environmental perturbations alter stem cell function and fate, leading to dysfunctional repair and remodeling.
Aberrant stem cell reprogramming in preterm infants and their antecedent diseases now support the notion that early alterations in stem cell function contribute to bronchopulmonary dysplasia (BPD) pathogenesis. New exciting preclinical data provide evidence that stem cell–based therapies reduce lung injury in BPD models. Among the cells being tested, mesenchymal stem cells have shown tremendous promise owing to their ability to replenish the endogenous stem niche, their immunomodulatory properties, and their availability. Clinical trials in several countries using mesenchymal stem cell–based therapies are now underway in preterm infants. This chapter discusses recent advances in lung stem cell biology, our current understanding of the impact of BPD on endogenous stem cells, and the application as well as challenges of implementing stem cell–based therapies for BPD.
Stem Cells
In the early 1900s the Russian histologist Alexander Maximow discovered that all blood cells have a common ancestral origin. Since then our knowledge of stem cell biology has grown exponentially, and stem cells are now known to be essential for organogenesis and tissue homeostasis. By definition, stem cells must have the ability to both replenish themselves through self-renewal and to differentiate into mature progeny. They may divide symmetrically or asymmetrically, giving rise to a stem cell and a more committed progenitor cell ( Fig. 21.1 ). They may also be classified as totipotent, pluripotent, or multipotent ( Fig. 21.2 ). Totipotent stem cells are capable of differentiating into all adult and embryonic tissues, including extraembryonic tissues such as trophectoderm. In mammals, only the zygote and the first cleavage blastomeres are totipotent. Pluripotent stem cells are capable of differentiating into derivatives of all three germ layers (ectoderm, mesoderm, and endoderm) and are typically derived from embryos at different embryonic stages of development. Multipotent stem cells are able to differentiate into multiple cell types of one lineage. The most prominent example remains hematopoietic stem cells, which are capable of differentiating into all cell types of the hematopoietic system. Stem cells may also be categorized as embryonic or adult stem cells. Embryonic stem cells are derived from blastocysts in the developing embryo and are pluripotent. Adult stem cells are found in tissues in specialized microenvironments. These cells are typically multipotent and following an asymmetric cell division, they produce a population of transit-amplifying progenitor cells. They may act as intermediates between dedicated stem cells and mature differentiated cells. Within tissues, there may also be facultative stem cells or progenitors, which are normally quiescent differentiated cells, but following injury, they may self-renew and give rise to other differentiated progeny.


Endogenous Lung Stem Cells
The postnatal lung contains stem cells capable of reconstituting the lung following injury ( Table 21.1 ) and these are predominantly facultative stem cells. These endogenous lung stem cells are limited in their differentiation potential, have potency restricted to lung cell lineages and topographically, are localized to specific anatomic regions and tissue micro-environments. Despite attempts to identify a dedicated lung stem cell, there is no clear evidence of such a cell. Instead, it is more likely that there are several niches of multipotent cells with the potential to reenter the cell cycle during injury. It is also conceivable that prematurity and its associated exposures dysregulate signaling pathways that modulate stem cell programming, leading to perturbation of these endogenous niches and dysfunctional repair.
Cell Type | Marker Genes | Location | Differentiation Potential | Role in BPD | References |
---|---|---|---|---|---|
Basal cells | Trp63, Krt5, Krt14, NGFR, Pdpn | Trachea | Self, ciliated, club | Unknown | |
Club cells | Scgb1a1, Cyp2f2 | Bronchiole | Self, ciliated | Unknown, killed by naphthalene | |
Variant club cells | Scgb1a1, Cyp2f2 low | Near neuroepithelial bodies | Self, ciliated, club | Unknown but survive naphthalene injury | |
BASCs | SP-C, Scgb1a1 | Self, club, ciliated, AEC2 | Unchanged in rodent hyperoxia-models of BPD but gives rise to AEC2 cells following bleomycin injury and increases after intratracheal MSC therapy in rodent hyperoxia BPD models | ||
Integrin α6β4 alveolar progenitors | Itgα6, Itgβ4 | Conducting airways, BADJ, alveolar wall | Self, club, ciliated, AEC2, AEC1 | Unknown but may contribute to alveolar repair following bleomycin-induced lung injury | |
Distal airway stem cells | Trp63, Krt5, Krt14, NGFR, Pdpn | Distal airways | Self, club, ciliated, AEC2, AEC1 | Unknown but migrate and give rise to alveolar cells following severe influenza | |
AEC2 | SP-C | Alveoli | Self, AEC1 | Proliferates in response to hyperoxia and gives rise to AEC1 cells |
Airway Candidate Stem Cells
Within the proximal gas-conducting system (the trachea and main bronchi) is a distinct population of undifferentiated basal cells expressing cytokeratins 5 and 14, transcription factor 63, and nerve growth factor that functions as bronchiolar stem cells. These basal cells self-renew and give rise to ciliated and club cells (formerly known as Clara cells) under steady-state conditions and following sulfur dioxide inhalation injury. Within the distal airways, lineage tracing experiments show that club cells self-renew and differentiate into ciliated cells. Evidence also points to different club cell populations with variable proliferative capacity and lineage potential. One club cell subpopulation is resistant to the toxin, naphthalene, which poisons the cytochrome P450 enzyme. These cells have been termed “variant club cells” and are located around the neuroendocrine bodies and at the bronchoalveolar duct junction. Variant club cells are quiescent in the steady state; however following naphthalene-induced injury they proliferate quite rapidly. The role of these cells in BPD is unknown.
Alveolar Candidate Stem Cells
Within the alveoli, substantial in vivo and in vitro evidence suggest that alveolar type 2 epithelial cells (AEC2) are the progenitors of alveolar type 1 epithelial cells (AEC1). AEC2 cells play a crucial role in replenishing the AEC1 population under both steady-state and injury conditions, and lineage tracing experiments reveal that AEC2 cells not only self-renew but also give rise to AEC1 progeny. In three-dimensional (3D) culture, AEC2 cells form alveolospheres containing cells that express both AEC2 and AEC1 cell markers. Emerging data also show different subpopulations of AEC2 cells with variable regenerative potential. In whole lung and primary cultures of adult rat AEC2 cells, there is a hyperoxia-resistant subpopulation of telomerase-positive AEC2 that expands in response to injury. AEC2 cells expressing high surface levels of telomerase but low epithelial (E)-cadherin are more proliferative and less likely to undergo hyperoxic damage compared with AEC2 cells that express high levels of E-cadherin.
More recently, rare populations of undifferentiated basally located distal airway stem cells expressing cytokeratin 5, transcription factor 63, and cytokeratin 14 have also been identified as potential alveolar progenitors. These cells originate from SOX2+ airway progenitors and are inactive under steady-state conditions, but they proliferate and migrate into the alveoli following severe influenza-induced lung injury, differentiating into both airway and alveolar lineages. Additional populations of multipotent epithelial stem cells that express the laminin receptor α6β4 have also been described. These cells are localized within the epithelium of the conducting airways and alveoli and have the capacity to give rise to AEC2 cells, suggesting the possibility that these multipotent distal airway stem cells may contribute to alveolar repair.
Other candidate alveolar progenitors include rare populations of epithelial cells located at the bronchoalveolar duct junction, so-called bronchoalveolar stem cells (BASCs). These cells express both surfactant protein C (SP-C) as well as secretoglobin (Scgb1a1), are resistant to naphthalene, and proliferate rapidly after naphthalene- and bleomycin-induced lung injury Although BASCs are unchanged in hyperoxia-induced lung injury and their role in lung development is unclear, BASCs are able to give rise to AEC2 cells following bleomycin-induced lung injury, albeit to a minor degree. In specialized culture media, BASCs give rise to cells that express pro-SP-C, aquaporin 5, as well as Scgb1a1, suggesting that these cells could give rise to cells in the conducting as well as gas exchange portion of the lung. However, more research is needed to elucidate the regenerative capacity of these alveolar progenitor cells in BPD, their interactions with cells in the lung vasculature and mesenchymal compartment, and the molecular mechanisms that drive these alveolar progenitors toward quiescence or activation.
Lung Mesenchymal Stem Cells
Originally characterized in 1968 by Friedenstein and colleagues, mesenchymal stem cells (MSCs) were first described as a population of BM stromal cells that were adherent, fibroblastic in appearance, and clonogenic. Because there are no specific cell markers for MSCs, the International Society for Cellular Therapy established minimal criteria for defining MSCs: (1) adherence to plastic under standard tissue culture conditions; (2) expression of cell surface markers, CD105, CD90, and CD73, and no expression for HLA-DR, CD79α, CD45, CD34, CD14, CD19, and CD11b; and (3) the capacity to differentiate into osteoblasts, chondroblasts, and adipocytes under appropriate in vitro conditions.
Although these criteria were mainly used to describe BM-derived MSC, emerging data now suggest that most organs have endogenous MSC populations, albeit with varying functionality, differentiation potential, and phenotype, depending on micro-environmental cues. MSCs have been isolated from fetal and adult lungs based on their adherence to plastic, expression of adenosine-binding cassette G (ABCG2), Hoechst 33342 dye efflux, and their osteogenic, chondrogenic, and adipogenic differentiation potential. Compared with BM-MSCs, lung-derived MSCs seem to be constitutively more prone to epithelial differentiation and have a distinct pattern of Hox gene expression implicated in lung development. Although lung and BM-MSCs reduce elastase injury to the same extent, lung MSCs express more intercellular adhesion molecule 1 (ICAM-1), platelet-derived growth factor receptor alpha (PDGFRα), and integrin α2 than BM-MSCs, conferring potential differences in MSC adherence, migration, and invasion. Moreover, lung MSCs are now believed to be a part of a diverse population of mesenchymal progenitors directly involved in regulating the growth and function of epithelial progenitor cells.
The role of lung MSCs in BPD is still being clarified. Lung MSCs are decreased in the lungs of newborn rodents exposed to hyperoxia, yet increased MSCs in the tracheal aspirate of preterm infants predicts an increased risk for BPD by more than 25-fold. Moreover, MSCs isolated from the lungs of preterm infants differentiate into myofibroblasts under the influence of the pro-fibrotic factor, transforming growth factor beta. Similarly, MSCs isolated from human lung allografts undergo pro-fibrotic differentiation in response to cytokines associated with bronchiolitis obliterans, but a significant decrease in lung MSCs is evident in the lungs of rodents with bleomycin-induced pulmonary fibrosis and replacement of lung MSCs attenuates fibrosis. One potential explanation for these apparent contradictory findings is the presence of different subpopulations of lung MSCs with variable epithelial-supportive capacity and myofibroblastic differentiation potential. Conceivably, depletion of lung MSCs may interfere with the endogenous regenerative response; conversely, lung microenvironmental cues may influence MSC behavior and function, potentially driving them toward a more dysfunctional fibrotic phenotype.
Lung Endothelial Progenitors
Endothelial progenitors (EPCs) were first described by Asahara et al. in 1997. This group described a population of peripheral blood mononuclear cells that could differentiate into endothelial cells. These cells expressed the hematopoietic stem marker, CD34, as well as the endothelial cell marker, vascular endothelial growth factor receptor 2 (VEGFR2), and were shown to contribute to physiologic and pathologic neovascularization. Since then various different markers have been used to identify this population, and although still marred with controversy, current data suggest that there is a hierarchy of EPCs based on their proliferative potential. One subset appears early in culture, displays endothelial markers, but does not form vessels in vivo. These cells exert mainly paracrine effects on endothelial cells. Another subset, termed endothelial colony-forming cells (ECFCs), gives rise to colonies 7 to 21 days after plating, has robust proliferative potential, and forms vessels when transplanted in vivo. These cells can not only be isolated from umbilical cord and aorta vessel walls, but recent evidence also points to similar cells within the pulmonary microvasculature. This is intriguing given the importance of angiogenesis in lung development and repair. Indeed, prior studies have linked circulating EPC quantity with adult lung disease outcomes. Patients with end-stage chronic lung disease have reduced circulating EPCs and patients with idiopathic pulmonary fibrosis showed a marked EPC depletion, particularly when pulmonary hypertension is evident. Interestingly, ECFCs isolated from neonatal rats with hyperoxia-induced BPD-like lung injury proliferate less and form fewer capillary-like networks, suggesting that functional deficiency in ECFCs may contribute to BPD pathogenesis. This is further supported by evidence of decreased and dysfunctional cord ECFCs in preterm infants in whom BPD subsequently develops.
Cell Therapies for Bronchopulmonary Dysplasia
Evidence from Preclinical Studies
The recent insights into stem cell biology have unraveled the therapeutic potential of stem cells. Specifically, MSCs have attracted much attention because of their ease of isolation, culture, and expansion; allogeneic use; and pleiotropic therapeutic effects. The latter is of particular interest for a multifactorial disease such as BPD, in which multiple pathophysiologic mechanisms contribute to impaired alveolar and lung vascular development.
Early proof-of-concept studies in neonatal rodents exposed to hyperoxia showed that rodent BM-MSCs administered intravenously (IV) or intratracheally (IT) prevent the arrest in lung vascular and alveolar growth in this model ( Fig. 21.3 ). An interesting source of MSCs, especially when treating neonatal diseases, is represented by perinatal tissue. The placenta and umbilical cord are usually discarded after birth, yet they contain a large number of various cells, including MSCs, with therapeutic potential and can be harvested without harm to the mother or the newborn. After the above-mentioned proof-of-concept studies, MSCs derived from the umbilical cord (UC) Wharton jelly and from the umbilical cord blood (UCB) have been studied extensively in experimental BPD models. Similar to the therapeutic benefit reported for BM-MSCs, UC- and UCB-derived MSCs demonstrated comparable effects in hyperoxia-induced neonatal lung injury in rodents : improved alveolar and lung vascular structure and even restoration of alveolar growth after established lung injury, attenuation of lung fibrosis, reduced lung inflammation, and improved exercise capacity. The therapeutic benefit persisted into adulthood with no evidence of tumor formation or adverse effects on lung architecture. Since then numerous investigators have attempted to optimize the use of MSCs by exploring the best timing, dose, and route of administration as outlined further in the following text. A consistent finding, however, was the low rate of lung engraftment of MSCs. Increasing evidence suggests that MSCs act via a paracrine mechanism to protect the developing lung from injury. Table 21.2 provides a summary of the preclinical evidence.

Cell Population | Cell Dose | Timing of Administration | Model | Route of Delivery | Effects of Therapy | Suggested Mechanisms of Repair | References |
---|---|---|---|---|---|---|---|
Mouse BM MSCs or CM | 5 × 10 4 | Postnatal day (P)4 | Neonatal rats in hyperoxia P1–P14 | Intravenous (IV) | CM have more pronounced improvement in alveolar and vascular structure than MSCs | Paracrine-mediated | |
Rat BM MSCs | 1 × 10 5 | P4 (prevention) P14 (treatment) | Neonatal rats in hyperoxia P1–P14, studied on P21 (prevention) or P45 (treatment) | Intratracheal (IT) | Early but not late MSC administration preserves lung architecture and survival. Both early and late administration improve exercise capacity | Paracrine-mediated | |
Human UCB MSCs | 5 × 10 3 5 × 10 4 5 × 10 5 | P5 | Neonatal rats in hyperoxia Birth–P14 | IT | Higher-dose MSCs have more pronounced effects on alveolar structure | Modulation of host inflammatory and antioxidant responses | |
Male rat BM MSCs or CM | 2 × 10 6 | P9 | Neonatal rats in hyperoxia P2–P16; evaluated at P16, P30, and P100 | IT | MSC and CM similarly improve lung architecture at P16 and P30; however, MSCs have more pronounced effects than CM at P100 | Reduced inflammation | |
Male and female rat BM MSCs | 1 × 10 6 | P7 | Neonatal rats in hyperoxia P2–P21 | IT | Improved alveolar structure, increased vascular density, reduced pulmonary hypertension (PH) and remodeling | Female MSCs have greater antiinflammatory and pro-angiogenic effects than male MSCs | |
Human UCB MSCs | 5 × 10 5 (IT) 2 × 10 6 (IV) | P5 | Neonatal rats in hyperoxia Birth–P14 | IT or IV | IT administration improves body weight and is better than IV in improving alveolar structure | IT administration has greater MSC retention and is better than IV in reducing inflammation and apoptosis | |
Human UCT MSCs | 0.1 × 10 6 0.5 × 10 6 1 × 10 6 | P5 | Newborn SCID mice in hyperoxia Birth–P7; evaluated 8 weeks after MSC transplantation | Intranasally or IP | High-dose IP MSCs restore lung compliance, elastance, and pressure-volume loops. Intranasal or low-dose MSCs have no significant effects | Paracrine-mediated | |
Human UCB MSCs | 5 × 10 5 | P3 (early) P10 (late) P3 + P10 | Neonatal rats in hyperoxia 90% for 2 weeks and 60% for 1 week | IT | More pronounced improvement in alveolar structure, oxidative stress, inflammation with early compared with late MSCs | Paracrine-mediated | |
Human cord PCs or UCB MSCs | 3 ×10 5 (early) 6 × 10 5 (late) | P4 (early) P14 (late) | Neonatal rats in hyperoxia P4–P14; evaluated at P22 (prevention) or P35 (regeneration) | IT | Both early and late MSCs and PCs improved alveolar structure and lung function. No tumors evident at 6 months | Paracrine-mediated |
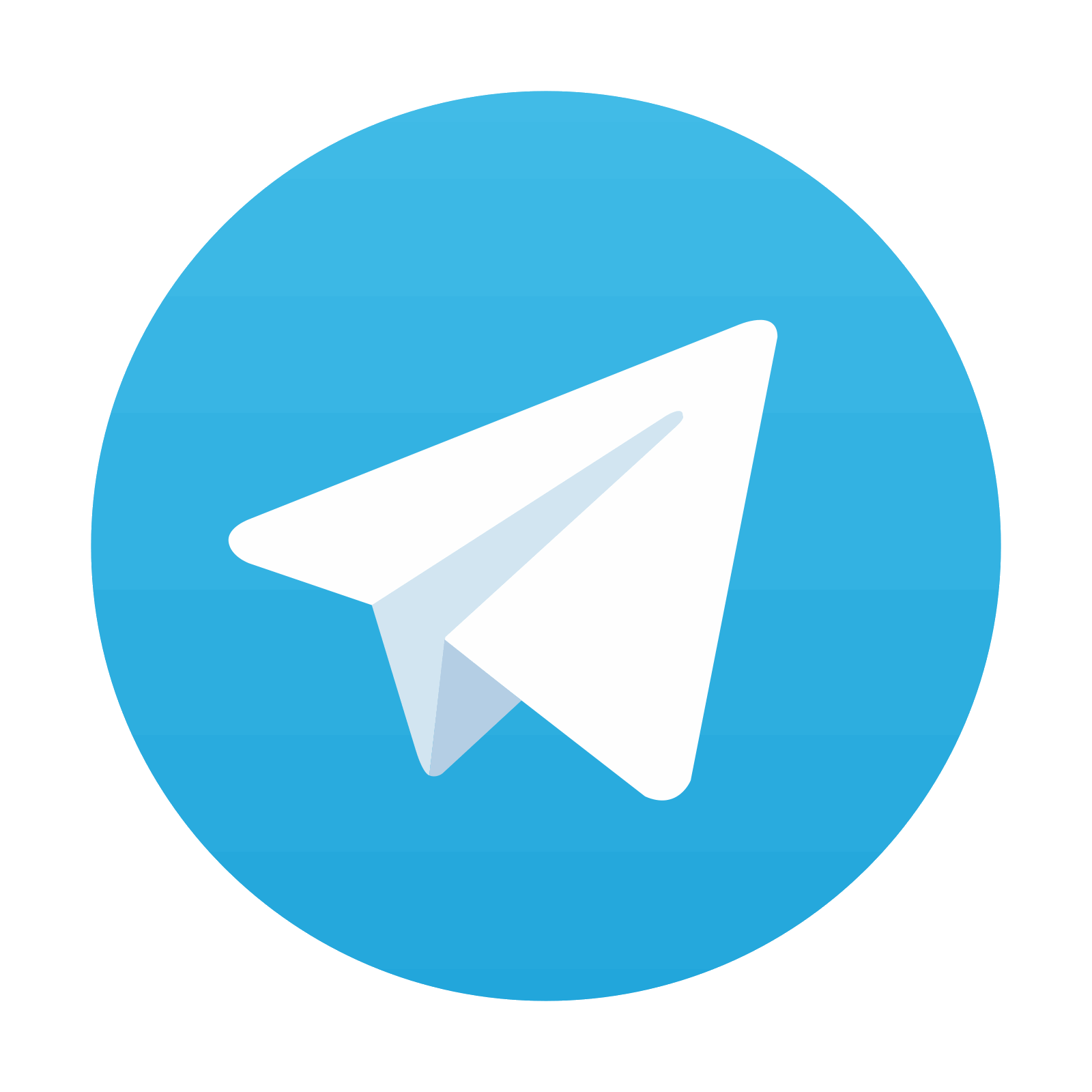
Stay updated, free articles. Join our Telegram channel
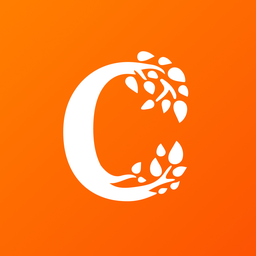
Full access? Get Clinical Tree
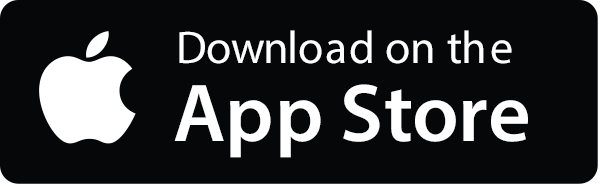
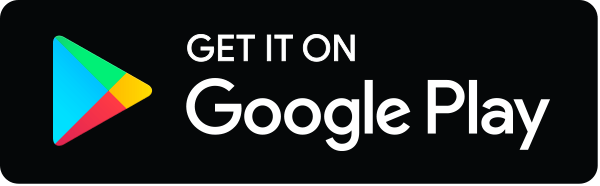