Cardiovascular Drugs
Eli Zalzstein
Rafael Gorodischer
Drugs Used in the Management of Congestive Heart Failure
General Considerations
Heart failure is defined as the pathophysiologic state wherein the heart is unable to pump adequate volume of blood and oxygen at a rate appropriate to the body’s metabolic demands. Heart failure is now considered more a cardiocirculatory disorder than simply a disease of the heart. Typically, it is characterized in hemodynamic terms and is considered to occur when changes take place in one or more of the determinants of cardiac output, including preload, afterload, contractility, and heart rate. Depression of the intrinsic contractile performance of the cardiac muscle is typical for myocardial and for ischemic heart disease. Structural defects of the heart or great vessels result in abnormal loading conditions; they lead to heart failure even when the muscle contractility is normal. Large left-to-right shunts, valvular insufficiency, and atrioventricular (AV) malformations lead to volume overload, whereas obstructive lesions such as severe aortic stenosis may lead to pressure overload and failure. Extracardiac conditions like hyperthyroidism, anemia, and severe malnutrition may result in high cardiac output failure secondary to unbalanced demand supply of the heart.
As a reaction to heart failure the body activates compensatory mechanisms to maintain adequate systemic oxygen delivery. Renal salt and water retention and augmentation of cardiac preload relationship maximize cardiac output through the Frank–Starling law. Catecholamine release is another compensatory mechanism, which augments myocardial contractility and heart rate. Myocardial hypertrophy leads initially to decreased ventricular wall stress but finally may deteriorate to decreased contractility. All these compensatory mechanisms contribute initially to maintain pump function but finally lead to progressive deterioration in cardiac performance and congestive heart failure.
The progressive impairment of ventricular function is the result of structural and conformational changes of the heart, with deleterious remodeling due to hypertrophy and fibrosis. Increased systemic and intracardiac neurohormone concentrations are a main factor in promoting cardiac remodeling. In addition, neurohormones act as growth factors for cardiac myocytes and fibroblasts. This new knowledge has led to the use of agents that block the renin–angiotensin–aldosterone system and the sympathetic nervous system to inhibit, and even reverse, cardiac remodeling (1).
The heart of the young infant responds in a more limited fashion to inotropic agents than that of the older child or adult (2,3,4,5,6). This is due to the biologic immaturity and restricted functional reserve of the young heart as well as the different pathogenesis of cardiac decompensation of the myocardium in infants with left-to-right shunts (7,8), a lower ratio of active myofilaments to noncontractile elements (9), greater stiffness of the ventricle (10,11), underdeveloped cardiac sympathetic nerves (12,13), and higher cardiac output per unit surface area (14). Differences in electrolyte concentration and in a number of metabolic reactions have also been described in the newborn myocardium (15,16).
Traditionally, digitalis glycosides and diuretics have been key drugs in the management of congestive heart failure. Concern with the low therapeutic index of digitalis led to the increased use of natural and synthetic catecholamines in acute situations and to the introduction of novel inotropic agents. The myocardium of many infants with congenital heart defects may already be working at the peak of its contractile force; thus, it may be unable to sustain adequate blood flow because of volume overload (due to left to right shunting in patent ductus arteriosus and ventricular septal defect) or pressure overload (in obstructive lesions) (2,7,8). The use of systemic vasodilators reduces cardiac workload in infants and children in heart failure.
Therapy has shifted from purely hemodynamic manipulation to include neurohumoral modulation. Therapeutic classes that have emerged in the last decades include angiotensin-converting enzyme (ACE) inhibitors, spironolactone, β-blockers, β-type natriuretic peptide (nesiritide), and triiodothyronine.
Digoxin
Digoxin is the most commonly used cardiac glycoside, has been studied more than other preparations, and is the
glycoside recommended for routine use in pediatric patients. Objective data on its efficacy in the treatment of congestive heart failure have been provided in adults with normal sinus rhythm (17).
glycoside recommended for routine use in pediatric patients. Objective data on its efficacy in the treatment of congestive heart failure have been provided in adults with normal sinus rhythm (17).
The main action of digitalis is to increase the force of myocardial contraction. The positive inotropic effect in a patient with heart failure results in slowing of cardiac rate, disappearance of gallop rhythm, improved tissue perfusion, diuresis, relief of edema, and decreased venous pressure and heart and liver size. Cardiac glycosides also affect the metabolism, conduction, refractory period, excitability, and automaticity of the myocardium. In addition, they have extracardial hemodynamic effects such as arteriolar and venous constriction, both by direct action on vascular smooth muscle and by neural effects.
Mechanism of Action
Digitalis glycosides bind to multiple sites in many cells, including those of the myocardium (18,19,20,21). The pharmacologic actions of digitalis on the heart, however, seem to be due to surface interactions in the cardiac sarcolemma and are not correlated with the total tissue uptake of the drug (21,22,23,24).
The most specific action of cardiac glycosides seems to be the well-known inhibition of the Na+, K+ exchange pump of the cell membrane. Intimately related to the Na+ pump is the Na+, K+-ATPase which is located in the cardiac sarcolemma; it is inhibited by cardiac glycosides. Digitalis is postulated to enhance contraction by increasing the influx of Ca2+ as a result of inhibition of the Na+, K+-ATPase system.
A large body of evidence supports the hypothesis that the Na+, K+-ATPase is the receptor for cardiac glycosides (25). However, some facts do not fit this theory: The positive inotropic effect of cardiac glycosides is terminated by washing, whereas the inhibition of Na+, K+-ATPase persists; the kinetics of the inhibition of the Na+, K+-ATPase and of the positive inotropic effect are different, and the therapeutic concentrations of free digitalis in plasma are too low to evoke any inhibition of the Na+ pump in the heart (26,27).
Digitalis glycosides also influence cardiac function indirectly, by their action on the vagal nerve. In addition to improving hemodynamics, digitalis glycosides also have significant neurohormonal modulatory effects and reduce plasma renin and norepinephrine concentrations. Digoxin modulates the increased sympathetic autonomic activity, probably by improving arterial baroreceptor reflexes (28,29).
Absorption
Digoxin is available in both oral and parenteral forms. Gastrointestinal absorption varies from patient to patient and results in a lower serum concentration than does parenteral administration. In some patients, bioavailability of digoxin is affected by intestinal flora (30). P-glycoprotein, present in intestinal cells, pumps digoxin back into the intestinal lumen and thereby limits its absorption (31,32). Drugs such as quinidine, verapamil, spironolactone, and dipyridamole inhibit P-glycoprotein and increase digoxin bioavailability (33,34).
Distribution and Plasma Protein Binding of Digoxin
The affinity of digoxin for plasma proteins is low both in adults and in newborn infants. The apparent volume of distribution of digoxin in infants and in children is much greater than in adults (35,36,37,38). The affinity for digoxin varies in different tissues and is greatest in the ventricular myocardium (36,39). A linear relationship exists between myocardium and serum concentration over a wide range of concentrations (37,39,40). Greater myocardial and erythrocyte concentrations have been found in children during digitalization than in the course of chronic administration of digoxin (37,40).
Endogenous Digoxin-Like Substances
Endogenous digoxin-like substances (EDLS) have been determined in the serum of newborn infants, pregnant women, and patients with hypertension or renal and hepatic disease (41,42,43,44). EDLS may interfere with the clinical interpretation of serum concentrations during digoxin therapy. Newborn infants not treated with digoxin and not exposed to it in utero may have measurable concentrations of the glycoside within the “therapeutic” range. Concentrations tend to decrease with increasing gestational age.
Most digoxin assays do not have the specificity to fully distinguish EDLS from exogenous digoxin, although EDLS do not possess the same therapeutic properties as the drug. The resultant artificial elevation of a reported digoxin level or a completely factitious level in a patient not taking the drug can have pharmacokinetic and clinical consequences (45,46).
Cross-reactivity with EDLS may be avoided with a high-performance liquid chromatography assay. Although highly specific, the high-performance liquid chromatography assay is not practical for routine clinical use and is less sensitive as compared with the fluorescence polarization immunoassay (47). A microparticle enzyme and the TDx Digoxin fluorescent polarization immunoassay reportedly minimize EDLS cross-reactivity (48,49).
Renal Excretion
Digoxin is excreted largely unchanged in the urine. A linear relationship exists between the renal clearance of digoxin and creatinine; in adult subjects, these two values are usually identical (50,51,52). It is subjected to active tubular secretion and to some degree of tubular reabsorption (50,51).
Renal clearance of digoxin is low during the first weeks of life and increases progressively until adult values are attained at about 5 months of age (53). At any given age, however, renal clearance of digoxin in infants is greater than the simultaneously determined creatinine clearance (53,54). The rate of digoxin clearance is age dependent and correlates with renal P-glycoprotein expression (55). Renal excretion of digoxin is impaired in patients with renal failure (56). Diuretics such as furosemide do not significantly affect renal excretion of digoxin (57).
Clinical Efficacy of Digoxin in Fetuses, Infants, and Children
Whereas the efficacy of cardiac glycosides in supraventricular arrhythmias is well established (58), similar evidence for efficacy in congestive heart failure in children has been controversial; inotropic agents may not improve hemodynamics substantially (59,60,61,62). Because cardiac failure stems from a congenital malformation in most of these cases (e.g., ventricular septal defect or persistent ductus arteriosus), the already hyperactive and presumably healthy myocardium may not benefit from digitalis.
Most infants and children with congestive heart failure are treated with digoxin, diuretics, and other drugs, which makes it difficult to assess the net effect of the glycoside in a given patient. Only about half of infants with ventricular septal defect alone may benefit from digoxin therapy (60,61,63,64,65,66). Studies in adults indicate that digoxin does have a significant salutatory effect in patients with heart failure (17,30,67).
In the treatment of supraventricular tachycardia, digoxin is efficacious because of both direct and indirect (autonomic) effects. It slows the rate of the sinus node through the vagal nerve and increases the refractory period, thus abolishing reentry of supraventricular tachycardia. Because protracted periods of fetal tachyarrhythmias (supraventricular tachycardia, atrial flutter, and atrial fibrillation) may cause congestive heart failure and fetal death, transplacental therapy with digoxin as well as other antiarrhythmics (e.g., quinidine, verapamil, amiodarone) is given. Maternal digitalization appears to be the treatment of choice in such cases, relying on the excellent transplacental distribution of the glycoside. Digoxin is also used in the treatment of fetal congestive heart failure with sinus rhythm (68).
Digoxin Toxicity
A substantial proportion (up to 25%) of hospitalized adults receiving digoxin experience some degree of adverse effects related to the drug (69). Although similar figures are lacking in pediatric patients, there is a clinical impression that toxicity is not as common in children, but large studies in infants and children are not available. Digoxin toxicity in infants is more common with loading (“digitalization”) doses than during maintenance therapy. A significant cause of digoxin toxicity is unintentional therapeutic error. The arrhythmias induced by digitalis are more likely to occur and are more severe in patients with cardiac disease. Hypokalemia, hypocalcemia, hypomagnesemia, and hypoxia are likely to increase the risk of toxicity; kaliuretic diuretics, which are often coadministered with digoxin, are a common reason for its toxicity.
The effective antidote for digoxin toxicity is a preparation of Fab fragments from antidigoxin antisera. The neutralizing dose of Fab fragments is based on the digoxin dose or the total body digoxin burden.
Digoxin Dose
Individual response to the drug varies, making it necessary to adjust the dosage according to the patient’s response. Digoxin therapy is most effective when the medication is given orally (Table 42.1). A digitalizing dose may be given over the first 24 hours, with one-half of the total digitalizing dose given initially and two one-quarter doses of the total digitalizing dose at 6- to 8-hour intervals following the previous dose. Oral maintenance therapy is usually begun 12 hours after the last digitalizing dose. When early signs of heart failure are present, the maintenance dose may be started without the digitalizing dose, although the full therapeutic effect will not be reached for 4 to 7 days. The advantage of this last approach is that it requires only one dosage calculation and decreases the likelihood of dosage errors or acute toxicity. Patients with inflammatory disease of the myocardium and in the postoperative state often have an enhanced sensitivity to the drug, which requires reducing the administered dose. The parenteral dose is generally calculated to be 25% less than the oral dose. Intravenous digoxin therapy can be used in instances of acute, severe heart failure. However, other forms of parenteral inotropic support are available that maybe more efficacious and safer to use.
Table 42.1 Digoxin Dosage Schedule | |||||||||||||||||||||
---|---|---|---|---|---|---|---|---|---|---|---|---|---|---|---|---|---|---|---|---|---|
|
Adverse Effects
Common adverse effects of digitalis include the heart (sinus bradycardia, A-V block, ventricular tachycardia, and fibrillation) and other systems: the gastrointestinal (nausea, vomiting, anorexia, diarrhea), the central nervous (dizziness, headache, malaise, pain, alterations in vision, aphasia, delirium), and others (i.e., gynecomastia).
Drug Interactions
Drugs coadministered with digoxin may interfere with the disposition of cardiac glycosides and cause potentially toxic serum concentrations (70). Quinidine, spironolactone, verapamil, amiodarone, and carvedilol (71) may elevate the serum concentration of digoxin and cause toxicity. Those drugs may inhibit the tubular secretion of digoxin, as they decrease its renal clearance without affecting the glomerular filtration rate. Whenever those drugs are coadministered with digoxin, the dose of the cardiac glycoside should be decreased, with careful monitoring of its serum concentrations.
Therapeutic Monitoring of Digoxin Therapy
In principle, digoxin could be a good candidate for monitoring therapy by measuring its serum concentration because it has a low therapeutic index, its effect is not easily measurable during routine therapy, and a poor correlation exists between dose and serum concentration. However, a clear association between serum concentration and toxic and nontoxic effects does not exist, and assays for its measurement are not sufficiently specific. As mentioned, the existence of EDLS in the newborn may lead to spurious elevation of digoxin measurements. Still, serum digoxin concentrations correlate better than digoxin doses with its pharmacologic effect (74). Extremely high or immeasurable levels help in the decision of whether the patient has toxic symptoms.
Most authorities regard the therapeutic range of digoxin to be 0.5 to 2.0 ng per mL. With digoxin concentrations above 2 ng per mL there is an increased risk of digitalis toxicity. However, there is a wide “gray zone” of levels that may be toxic in one individual and nontoxic in another. Even at serum concentrations above 5 ng per mL, about one-third of pediatric patients may not show symptoms or signs of toxicity (75). A variety of clinical conditions, including hypokalemia, hypocalcemia, hypomagnesemia, and chronic heart disease, may increase the risk of toxicity of digitalis glycosides.
Little information exists on the correlation of serum concentrations of digoxin with inotropic effects; more data exist on the relationship between its serum concentrations and its antiarrhythmic effects. Some investigators have observed a good correlation, whereas others have not (76,77,78,79).
Optimal hemodynamic, neurohormonal, and clinical effects may be achieved in adults with a dose that results in a plasma concentration of about 0.7 ng per mL; no additional benefits are seen with concentrations above 1 ng per mL (30).
Measurement of serum digoxin concentrations is recommended in cases of suspected toxicity, in suspected accidental or suicidal digoxin ingestion, in high-risk patients (renal failure, following cardiac surgery), and in suspected underdigitalization (lack of compliance, inadequate dose, or gastrointestinal disturbances) (80).
Table 42.2 Use of Selected Inotropic Agents | |||||||||||||||||||||||||||
---|---|---|---|---|---|---|---|---|---|---|---|---|---|---|---|---|---|---|---|---|---|---|---|---|---|---|---|
|
Other Inotropic Agents
Catecholamines
Adrenergic agents exert a positive inotropic effect by acting directly on βl-adrenergic receptors of the myocardium. They may be used temporarily in the management of congestive heart failure (except in congestive failure due to obstructive lesions) until a more permanent therapy is established. Their clinical use is limited by their positive chronotropic action and tendency to exacerbate cardiac arrhythmias. There is no clear relationship between plasma catecholamine concentration and effect. Their actions in the heart include interaction with plasma membrane G protein, stimulation of adenyl cyclase, increased intracellular concentration of cyclic adenosine monophosphate (AMP), and Ca2+ delivery to cardiac contractile proteins, mediated by cyclic AMP-dependent protein kinases (81). The function of adrenergic receptors is affected by catecholamine as well as by tissue perfusion and pH. Clinical use of these agents is summarized in Table 42.2.
Epinephrine
Epinephrine (adrenaline), the major hormone secreted by the adrenal medulla, is a potent stimulator of both α- and β-adrenergic receptors and exerts complex effects on various organs. Following subcutaneous or slow intravenous administration, it increases the force of myocardial contraction and the heart rate, with a resultant increase in cardiac output and systolic pressure; diastolic pressure usually falls because of its effect on β2-adrenergic receptors of skeletal muscle vasculature. The increased work of the heart is achieved at the expense of greater oxygen consumption and decreased cardiac efficiency. In the kidneys, epinephrine increases vascular resistance and decreases plasma flow. Epinephrine also induces automaticity of the myocardium, and its intravenous administration may cause cardiac arrhythmias.
The effects of epinephrine infusion on regional blood flow are of concern in the management of critically ill children. The pulmonary vascular bed contains α- and β2
receptors, and pulmonary vasoconstriction (α stimulation) or vasodilation (β2 stimulation) can be expected, depending on the infusion rate, the duration of exposure to epinephrine, the presence of failure hypoxia, and the preexisting pulmonary vascular tone (82,83,84,85,86,87). At low and medium doses (less than 0.5 to 0.8 μg per kg per minute) epinephrine decreases pulmonary vascular resistance (PVR) and increases pulmonary blood flow; ventilation/perfusion mismatch may result. Higher doses appear to raise PVR if the preinfusion PVR was normal. However, even under these circumstances systemic vascular resistance is increased more than PVR, so that in patients with intracardiac defects, epinephrine infusion would favor left-to-right shunting. Conversely, if the preinfusion PVR was elevated by either hypoxia or sepsis, even high-dose epinephrine administration (1 to 3.5 μg per kg per minute) may yield predominantly β-adrenergic stimulation and pulmonary vasodilation (88,89,90).
receptors, and pulmonary vasoconstriction (α stimulation) or vasodilation (β2 stimulation) can be expected, depending on the infusion rate, the duration of exposure to epinephrine, the presence of failure hypoxia, and the preexisting pulmonary vascular tone (82,83,84,85,86,87). At low and medium doses (less than 0.5 to 0.8 μg per kg per minute) epinephrine decreases pulmonary vascular resistance (PVR) and increases pulmonary blood flow; ventilation/perfusion mismatch may result. Higher doses appear to raise PVR if the preinfusion PVR was normal. However, even under these circumstances systemic vascular resistance is increased more than PVR, so that in patients with intracardiac defects, epinephrine infusion would favor left-to-right shunting. Conversely, if the preinfusion PVR was elevated by either hypoxia or sepsis, even high-dose epinephrine administration (1 to 3.5 μg per kg per minute) may yield predominantly β-adrenergic stimulation and pulmonary vasodilation (88,89,90).
Few data exist on the pharmacokinetics of epinephrine in children (88). Plasma elimination half-life is very short (around 2 minutes).
Whereas noncatecholamine sympathomimetic amines act indirectly in the heart (through release of norepinephrine), epinephrine directly stimulates β1-adrenergic receptors of cardiac muscles and pacemaker and conductive tissue.
Epinephrine is administered only parenterally. After oral administration it is readily metabolized in the gut and the liver. It is readily absorbed by mucosa of the tracheobronceal tree, a route employed in cardiac resuscitation. It was shown to be safe as inhaled form in limited conditions as transient tachypnea of the new born (91). Epinephrine is rapidly inactivated by catecholamine-O-methyltransferase (COMT) and monoaminooxidase of the liver and other tissues, and its metabolites are excreted in the urine.
Toxic effects include restlessness, anxiety, fear, headache, tremor, pallor, dizziness, and palpitation. The major life-threatening toxic effect is the induction of ventricular arrhythmias. Interaction between epinephrine and halogenated hydrocarbon anesthetics may result in ventricular fibrillation. Tissue ischemia can occur as a consequence of peripheral vasoconstriction, especially with high rates of infusion.
Epinephrine for injection is a sterile solution of epinephrine hydrochloride in water. When administered by intravenous route the 1:1,000 solution should be diluted to 1:10,000 with saline; by endotracheal route the 1:1,000 solution is diluted with 3 to 5 mL of saline.
Norepinephrine
Norepinephrine is the neurotransmitter of sympathetic postganglionic fibers and of certain tracts in the central nervous system and constitutes 1/10 to 1/5 of the catecholamines in the adrenal medulla. It has β1– and α-adrenergic effects, but in contrast to epinephrine and isoproterenol, norepinephrine does not stimulate β2 receptors.
Intravenous infusion of norepinephrine causes an increase in peripheral resistance and a rise in systolic and diastolic blood pressure, with no change (or a decrease) in cardiac output; therefore, its use is limited to the management of hypotension and was demonstrated to be efficacious in extreme situations such as refractory septic shock (92). It has no indication in situations that require increased myocardial contraction force. In addition, norepinephrine may induce arrhythmias, tissue ischemia secondary to extreme vasoconstriction, and skin necrosis if cutaneous infiltration occurs. The mechanisms and extent of absorption, metabolism, and excretion are similar to those of epinephrine. It is administered by intravenous infusion at an initial dose of 0.1 μg per kg per minute and increased until the desired effect is obtained or up to 1 μg per kg per minute.
Isoproterenol
Isoproterenol (isoprenaline) acts on β1– and β2-adrenergic receptors, with minimal effect on α receptors. It is used in patients with depressed contractility and low cardiac output. It may reduce blood pressure, especially in patients with hypovolemia, but a reduction in systemic vascular resistance may be beneficial in some patients with elevated systemic resistance and low cardiac output. Isoproterenol may favorably reduce PVR in patients with pulmonary hypertension. Intravenous infusion of isoproterenol causes positive inotropic and chronotropic effects, decreased peripheral resistance and diastolic pressure, and increased renal blood flow. The resulting greater cardiac output may raise systolic pressure. Bradycardia due to AV block or sinus node dysfunction may be managed temporarily by infusing isoproterenol until more definitive therapy is available. Isoproterenol is rapidly absorbed when administered as aerosol, and it is metabolized primarily by COMT. Although less pronounced, its side effects are similar to those of epinephrine.
Dopamine
This sympathomimetic amine is a metabolic precursor of norepinephrine and epinephrine and acts as a central neurotransmitter. It acts on dopamine receptors of the brain and vascular beds in the kidney, brain, and coronaries. It has a positive inotropic effect on the myocardium resulting from direct stimulation of β1-adrenergic receptors and also from the effect of norepinephrine released by cardiac sympathetic nerve terminals. It has a less marked chronotropic effect than isoproterenol. At low therapeutic doses (2 to 5 μg per kg per minute), it has a predominant β1-adrenergic action and the increase in cardiac output parallels the increase in heart rate; at higher doses, dopamine has increasing α-adrenergic action. In addition, at low doses it causes renal vasodilation and promotes diuresis by stimulating renal D1– and D2-dopaminergic receptors and increases systolic pressure with little or no effect on diastolic pressure. Because it increases pulmonary artery pressure, it should be used with caution if at all, in patients with elevated PVR. At high doses (>10 μg per kg per minute), it stimulates arteriolar α-adrenergic receptors, causing vasoconstriction (including renal vasoconstriction) and hypertension.
In contrast to the response to isoproterenol, which is equal at all ages, the inotropic response to dopamine in the young animal increases with advancing age (89); this explains the greater dopamine requirements of young infants to obtain a therapeutic effect. This may be due to decreased
levels of releasable norepinephrine of the immature myocardium and/or greater dopamine clearance in infancy as compared to later in childhood (93).
levels of releasable norepinephrine of the immature myocardium and/or greater dopamine clearance in infancy as compared to later in childhood (93).
Dopamine does not cross the blood–brain barrier; it is metabolized by monoaminooxidase and COMT and therefore is effective only when given parenterally. No adverse effects have been recorded in infants, children, and adolescents with a dose of 0.3 to 25 μg per kg per minute (89). However, excessive dosing can cause side effects that represent enhanced sympathomimetic activity. Extravasations may cause ischemic necrosis. Phentolamine may be used when gangrene of the fingers or toes is feared following prolonged administration of the drug.
Dobutamine
Dobutamine is a synthetic β-sympathomimetic amine that has a potent inotropic action. It has little effect on heart rate in adults but significant chronotropic effects in young children. It is a mixture of L and D isomers, and its pharmacologic effects are explained by their different action on α1– (L isomer) and β1– and β2-adrenergic (D isomer) receptors. It does not stimulate renal dopamine receptors and has no effect on renal vasculature; low-dose dopamine may be added to the dobutamine infusion if increased renal cortical flow is desired. High doses of dobutamine may cause elevation of systemic arterial pressure. Increasing plasma concentrations correlate with improvement in cardiac function in adults with low output failure (93). The combination of dobutamine with dopamine improves cardiac performance at lower doses of each drug, preserving the renal vasodilatory effect of dopamine and reducing the potential for toxic reaction. Wide variability in drug clearance and in hemodynamic response requires individual titration of dobutamine therapy. It is effective even in preterm infants with low systemic blood flow (94).
Dobutamine plasma half-life is about 2 minutes, and its clearance in children is around 100 mL per kg per minute (95).
Newer Inotropic Agents
Some patients in cardiac failure remain symptomatic despite conventional therapy, including diuretics, angiotensive converting-enzyme inhibitors, and digoxin. Newer cardiotonics may be used in these patients which not only increase the contractility of the myocardium but also have vasodilating properties and the potential of a greater therapeutic index as compared with digoxin. Their inotropic effect is obtained by a mechanism different than inhibition of Na+, K+-ATPase, or β-adrenergic receptor stimulation (98,99). Data are available on these agents in the developing organism (5,100,101,102,103).
Phosphodiesterase Inhibitors
Inamrinone and Milrinone
Inamrinone (or amrinone) and milrinone are potent bipyridine derivatives that selectively inhibit cyclic AMP-specific cardiac phosphodiesterase and result in a positive inotropic effect, additive to that of digitalis. Infusion of inamrinone results in an increase in cardiac output with reduction in filling pressures and systemic vascular resistance. Heart rate is affected minimally by inamrinone at conventional doses. Inamrinone reduces pulmonary artery pressure without producing systemic hypotension in infants and children with intracardiac left-to-right shunt. Inamrinone may be effective in postoperative low-output states, and in this setting it has had the broadest application.
Both agents are well absorbed following oral administration, rapidly distributed in the body, and excreted in the urine. Inamrinone undergoes biotransformation in the liver to an N-acetyl derivative and other metabolites. The plasma elimination half-lives of inamrinone and milrinone in adults are around 4 and 1.5 hours, respectively. Plasma half-life of milrinone is about 3 hours in infants and 2 hours in older children (100). Neonates have a longer half-life and slower clearance of inamrinone than infants (101,102). The body clearance of both drugs is reduced in cardiac failure.
Inamrinone is metabolized by N-acetyltransferase, and its clearance is greater in fast acetylators (103). Clearance of milrinone is a function of age; its Vd was found to be 482 mL per kg in children after cardiac surgery (104).
Dosing regimens of milrinone have been designed for neonates (102,105). High dose of milrinone after pediatric congenital heart surgery reduces the risk for low cardiac output syndrome in infants and children (106) probably by a direct myocardial effect (107). Milrinone has been successfully administered in children with enterovirus-induced pulmonary edema (108) and in newborns with persistent pulmonary hypertension (109) and combined with nitric oxide, stabilizes pulmonary hemodynamics after Fontan operation (110).
Inamrinone acts rapidly following intravenous administration, whereas after oral dosing its effect peaks within 3 hours and lasts for 4 to 6 hours. The inotropic action of inamrinone is age dependent (5,111). Inamrinone and milrinone have direct vasodilator effects in newborn animals (112). Inamrinone has been shown to be useful in refractory septic shock (113). These bipyridines are efficacious in acute or decompensated cardiac failure, but their long-term use has resulted in serious side effects and increased morbidity in adults (114). Toxicity is related to dose and route of administration and includes gastrointestinal intolerance, hepatotoxicity, fever, and thrombocytopenia. Milrinone is better tolerated than inamrinone.
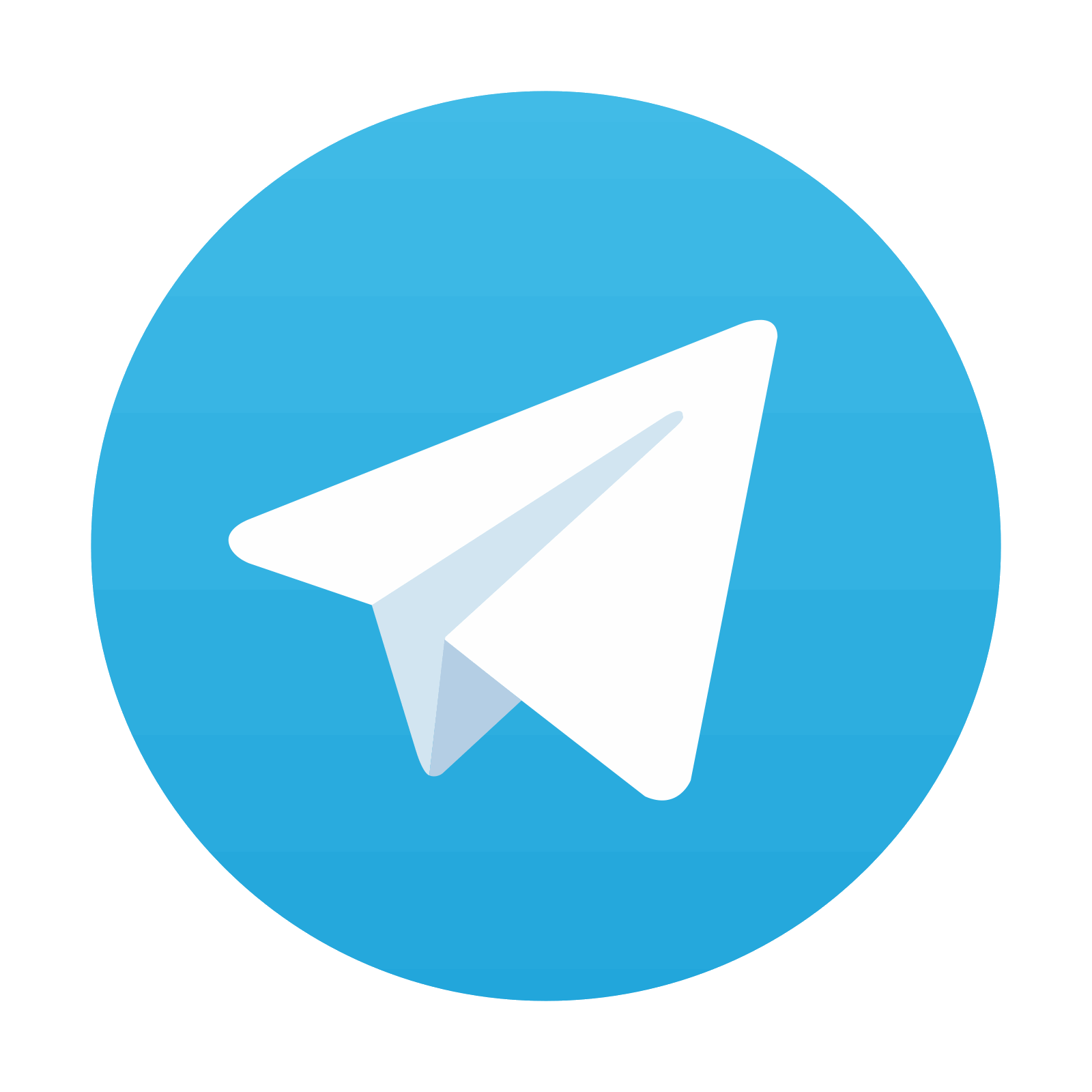
Stay updated, free articles. Join our Telegram channel
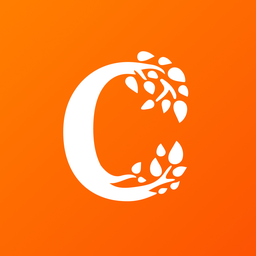
Full access? Get Clinical Tree
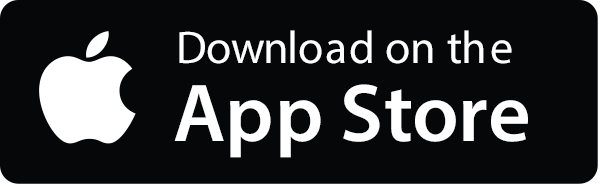
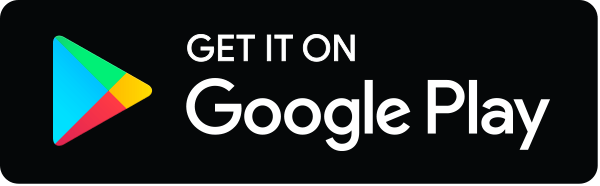
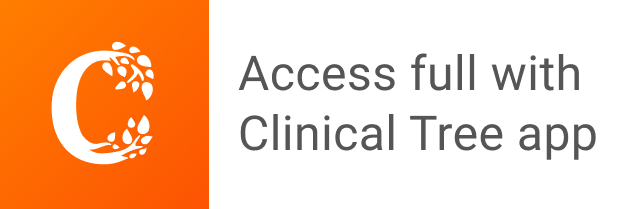