Cardiorespiratory Adjustments at Birth
Ruben E. Alvaro
Henrique Rigatto
Respiratory physiologists and physicians have long been interested in the respiratory and cardiovascular events that occur at birth. However, apart from occasional references to the pulmonary circulation, the fetal circulation only received serious consideration in the middle of the twentieth century, when it was recognized that dramatic changes in blood flow through the lungs occurred after birth.
The first detailed description of circulation in the mammalian fetus was provided by Harvey in 1628 (1). Although he correctly described blood flow from the inferior vena cava through the foramen ovale, he thought that the blood had to enter the pulmonary veins before returning to the left atrium. He was also perplexed by how the fetus survives in utero without the aid of respiration. The answer to the last question came in 1799, when Scheel noted light red blood in the umbilical vein and dark red blood in the umbilical artery in the fetal sheep, as well as darkening of that color when the pregnant ewe was asphyxiated (2). However, it was Zweifel, in 1876, who categorically stated that the placenta was the lung of the fetus, describing the presence of oxyhemoglobin in the umbilical blood before any breathing had occurred (3).
The conventional belief during the nineteenth century was that the fetal pulmonary blood flow progressively increased over gestation and that it was relatively higher in the fetus than after birth (4,5). It was not until the first part of the twentieth century that the right ventricular pressure was demonstrated to fall and pulmonary blood flow to increase after the establishment of breathing (6,7). It was only 50 years ago that Dawes and colleagues (8) demonstrated by direct measurements in fetal lambs that pulmonary blood flow increased when the lungs were ventilated with air. Over these past 50 years, the developmental changes in the pulmonary circulation and in its responses to stresses of hypoxia, and increases in pulmonary arterial pressure and blood flow, have become subjects of intense investigation (9).
It is well known now that during fetal life the placenta and not the lungs serves as the organ for gas exchange. Because of this, the normal fetal circulatory pattern is arranged very differently from that observed after birth and is quite satisfactory for survival in the womb. The placental circulation is in a parallel arrangement; that is, it receives blood from the descending aorta and drains blood to the systemic venous circulation. To accomplish this, the fetal circulation depends on a series of intra- and extracardiac shunts that allows the oxygenated blood to flow from the placenta to the systemic organs and for the deoxygenated blood to return to the placenta. This blood flow distribution allows the delivery of blood with the highest oxygen content (from the placenta) to the heart and brain and blood with lower oxygen content to the lower body and placenta. Because the lungs are not required for gas exchange, pulmonary blood flow is low (approximately 10% of the combined ventricular output), yet adequate for lung growth and development. Unique only to fetal life, the blood exiting the lungs has a lower saturation than does blood entering the pulmonary circulation, and although the lungs do not participate in gas exchange in utero, they are metabolically active, secreting liquid into the potential air spaces and synthesizing surfactant, a substance that is vital to achieve adequate ventilation at birth. The fetal lungs are also physically active in that they simulate breathing movements.
The transition from the placenta to the lungs at birth is accomplished by three main cardiopulmonary processes: (a) onset of breathing, resulting in lung expansion with concomitant decrease in pulmonary vascular resistance and increase pulmonary blood flow; (b) increase in blood oxygen content that further decreases pulmonary vascular resistance; and (c) loss of the placental circulation with resultant increase in systemic vascular resistance leading to the closure of the fetal cardiovascular shunts and transition from fetal to neonatal circulation. Thus, to establish the lungs as the site of gas exchange after birth, significant
changes in the cardiac and pulmonary circulation as well as the initiation of pulmonary ventilation must occur. Many abnormal maternal, placental and fetal conditions may interfere with this physiologic transition and compromise the newborn infant.
changes in the cardiac and pulmonary circulation as well as the initiation of pulmonary ventilation must occur. Many abnormal maternal, placental and fetal conditions may interfere with this physiologic transition and compromise the newborn infant.
The establishment of effective pulmonary ventilation at birth requires that the lungs develop to a stage where the alveoli can be inflated to provide adequate gas exchange. It also requires the lowering of the pulmonary vascular resistance to allow for the increase in pulmonary blood flow to accommodate the entire cardiac output. The successful transition also requires that the lung liquid volume be removed from the alveolar spaces and that surfactant material be secreted into the acinus to allow for satisfactory physical expansion of the lungs after the initial postnatal breaths. Adequate neurologic drive to generate and maintain spontaneous continuous breathing is essential to maintain ventilation postnatally.
The change to pulmonary ventilation at birth increases pulmonary venous oxygenation that serves to suppress active vasoconstriction of pulmonary vessels. The result is a tenfold increase in pulmonary blood flow and a rapid decrease in pulmonary vascular resistance. The removal of the placenta after constriction of the umbilical vessels in response to increased oxygenation, contributes to a rise in systemic vascular resistance. The decline in pulmonary vascular resistance below systemic values contributes to the closure of the foramen ovale and ductus arteriosus, establishing the adult circulatory patterns.
This chapter reviews some of the most important cardiorespiratory adjustments that occur at the time of delivery allowing the fetus to achieve a successful extrauterine transition.
PULMONARY ADAPTATION
Fetal Lung Fluid
During fetal life, the internal volume of the lungs is maintained by the secretion of liquid into the pulmonary lumen. This liquid expansion of potential air spaces is essential for the growth and the development of normal lung structure before birth, which, in turn, may influence lung function after birth (10).
TABLE 17-1 COMPOSITION OF LUNG LUMINAL LIQUID, AMNIOTIC LIQUID, AND PLASMA OF FETAL LAMBS | ||||||||||||||||||||||||||||||||
---|---|---|---|---|---|---|---|---|---|---|---|---|---|---|---|---|---|---|---|---|---|---|---|---|---|---|---|---|---|---|---|---|
|
The fluid in the fetal lung was for many years assumed to be aspirated amniotic fluid as a result of fetal breathing movements (11). In 1941, Potter and Bohlender (12) observed alveolar fluid in two human fetuses with malformations of the respiratory tract which blocked the entrance of amniotic fluid, thus establishing that the lung fluid was secreted, not inhaled. Experiments performed in other species confirmed that fetal pulmonary fluid was indeed generated within the lungs (13,14,15).
We know now that the fetal lung fluid is neither a mere ultrafiltrate of plasma nor aspirated amniotic fluid. Compared to plasma this lung fluid is rich in chloride and potassium, is significantly lower in bicarbonate and has similar sodium concentration. It is also quite different from amniotic fluid having much higher osmolality, Na+ and Cl– concentrations, and significantly lower K+, protein and urea concentration (Table 17-1) (10,16,17,18). This distinctive composition of the lung liquid changes very little during gestation (17,19). The high Cl– and the low protein content characteristics of the lung fluid result from active Cl– secretion and tight junctions between epithelial cells respectively.
It is not known exactly when this secretory activity begins, but already during the glandular stage of lung development, at about 3 months of gestation, the lung epithelium actively secretes fluid (20,21). By this time the epithelium has developed tight junctions, which are evident by morphologic examination and also by the low protein concentration present in the lung liquid in relationship to the plasma. In fetal lambs, the volume of lung liquid increases from about 5 mL/kg of body weight at midgestation (18) to about 30 to 50 mL/kg at term (18,22,23,24,25). The secretion rate increases from about 2 mL/ kg body weight at midgestation (18) to about 5 mL/kg at term (26,27). More recently, Pfister and associates (28) demonstrated that the lung liquid volume exhibited a plateau level in the near-term fetal sheep before it began to decline toward birth. They also observed that the rate of lung liquid secretion declined in two linear phases that commenced earlier than the changes in lung liquid volume (28). In fetal lambs, the amount of lung liquid in relation to lung weight remains
relatively constant at approximately 90% through much of the pregnancy (29,30). The lung liquid secretion decreases with increased luminal hydrostatic pressure induced by a prolonged obstruction of the fetal trachea and increases when luminal pressure falls below amniotic fluid pressure and when fetal breathing movements are abolished (24).
relatively constant at approximately 90% through much of the pregnancy (29,30). The lung liquid secretion decreases with increased luminal hydrostatic pressure induced by a prolonged obstruction of the fetal trachea and increases when luminal pressure falls below amniotic fluid pressure and when fetal breathing movements are abolished (24).
This liquid secreted by the fetal lungs flows intermittently up the trachea with fetal breathing movements. Some of this fluid is swallowed and the remainder contributes directly to the formation of amniotic fluid production, accounting for approximately 25% to 50% of the amniotic fluid turnover in the sheep fetus, with the rest being formed by the fetal urine (10). The mechanism by which amniotic fluid is not aspirated into the lungs was demonstrated by Brown and associates (31) in 1983, when they showed that the larynx acts as a one-way valve allowing only liquid outflow under normal circumstances. The continuous secretion of liquid by the lungs confronted with a flow impediment produced by the larynx and the amniotic fluid pressure creates a small but important positive intrapulmonary pressure, which is essential for normal growth and for the structural and biochemical maturation of the developing lung (10,29,31,32). Thus, in fetal sheep, unimpeded leakage of tracheal liquid decreases lung size by arresting pulmonary tissue growth, whereas prolonged obstruction of tracheal outflow leads to lung hyperplasia (32,33). Nardo and associates (34) showed that lung hypoplasia in fetal sheep can be considerably improved by short-term obstruction at the tracheal level. Conversely, pulmonary hypoplasia in humans can be observed in pathologic conditions such as diaphragmatic hernia, pleural effusion, or severe oligohydramnios (Potter syndrome) as a result of the compression of the fetal lungs and the decrease in their internal volume (35,36).
Congenital high airway obstruction syndrome (CHAOS) is a clinical condition caused by complete or near-complete obstruction of the fetal airway that results in elevated intratracheal pressure, distention of the tracheobronchial tree, and lung hyperplasia. The enlarged lungs may cause cardiac and caval compression leading to in utero heart failure manifested by ascites, hydrops fetalis, and placentomegaly (37,38).
The production of fetal lung liquid depends on a system of active ion transport across the alveolar type II cells of the pulmonary epithelium (10,39,40). Olver and Strang (41) demonstrated that lung liquid secretion is coupled with active transport of Cl– toward the pulmonary lumen, generating an electrical potential difference of -5 mV (lumen negative). This chloride secretion generates an osmotic gradient that causes liquid to flow from the microcirculation through the interstitium into the potential air spaces. This chloride secretion occurs through chloride channels in the apical membrane (alveolar side) and depends largely on chloride influx at a bumetanide-sensitive Na+-K+-2Cl– (NKCC) cotransporter system in the basolateral membrane (interstitial side) (42). Thus, Cl– enters the cell on a cotransporter linked with K+ and Na+ down the electrochemical potential gradient for Na+ generated by Na+-K+-adenosine triphosphatase (ATPase) in the basolateral membrane of the cell. Consequently, Cl– concentration increases inside the cell above its equilibrium potential, which provides an electrochemical gradient for Cl– exit across the luminal membrane of the epithelial cell through Cl– permeant ion channels (Fig. 17-1) (10). Addition of the loop diuretic bumetanide or furosemide (specific NKCC inhibitors) into the fetal lung liquid decreases fluid secretion by decreasing Cl– entry into the epithelial cell through the basolateral membrane.
Clearance of Fluid at Birth
To allow adequate postnatal gas exchange, pulmonary fluid, which is essential to fetal lung development, must be rapidly removed at birth in order (43,44,45). Thus the transition from intra- to extrauterine life requires the effective clearance of lung liquid to support air breathing and the conversion of the pulmonary epithelium in the distal air spaces from fluid secretion to fluid absorption. Disruption of this process has been implicated in several disease states, including transient tachypnea of the newborn (TTN) and hyaline membrane disease (44,46,47,48). Preterm delivery and cesarean section without prior labor result in excessive retention of lung fluid and may contribute to respiratory compromise in the newborn infant (47,49,50,51,52,53).
A complete understanding of the mechanisms by which fetal lungs are able to clear themselves of fluid is still lacking. It is clear now that the traditional explanations for fluid reabsorption which relied on Starling forces, lymphatic uptake and vaginal squeeze at the time of birth, can
only account for a very small fraction of the lung fluid clearance (54). More recently, several studies have strongly suggested that the primary mechanism of lung liquid reabsorption is the change in ion transport induced by catecholamines during labor (10,31,44,55,56,57). Although the mechanisms responsible for lung liquid clearance at birth develop gradually during the last part of pregnancy, the removal of lung liquid and the switch from fluid secretion to fluid absorption, triggered by events at birth, are probably quite rapid because in the human the functional residual capacity of the lungs rises to a near normal value of 25 to 30 mL/kg within 15 minutes after normal delivery (58).
only account for a very small fraction of the lung fluid clearance (54). More recently, several studies have strongly suggested that the primary mechanism of lung liquid reabsorption is the change in ion transport induced by catecholamines during labor (10,31,44,55,56,57). Although the mechanisms responsible for lung liquid clearance at birth develop gradually during the last part of pregnancy, the removal of lung liquid and the switch from fluid secretion to fluid absorption, triggered by events at birth, are probably quite rapid because in the human the functional residual capacity of the lungs rises to a near normal value of 25 to 30 mL/kg within 15 minutes after normal delivery (58).
Although there is disagreement about whether clearance begins prior to labor, or occurs entirely within labor, it is now clear that the vast bulk (>75%) of this liquid leaves the lung some time before normal term birth (59). In studies done in full-term fetal sheep, Pfister and associates (28) showed that the lung liquid volume began a gradual decline well before the onset of labor and it was mainly a result of a decrease in the secretion rate rather than active reabsorption. They also showed that this gradual decline was followed by a much more rapid decline within labor that was mainly a result of active reabsorption of fluid driven by active Na+ transport through the pulmonary epithelium. This last process is stimulated by the catecholamine surge that occurs just before the onset of labor (31). Other authors, however, suggest that lung liquid volume continues to rise until the day before labor (24,25,60).
As explained in the previous section, chloride secretion across the distal lung epithelium results in the production of lung liquid which is necessary for proper lung development in fetal life. In contrast, sodium absorption allows for fluid reabsorption and is critical for efficient oxygenation in the newborn.
The movement of sodium across the pulmonary epithelium from the alveolar lumen to the interstitium with subsequent absorption into the vasculature can be considered a two-step process. In the first step, sodium passively enters the apical membrane of the alveolar type II cell through amiloride-sensitive epithelial Na+ channels (ENaCs). Thus, intraluminal instillation of amiloride delays lung fluid clearance in fetal life (61,62,63,64,65,66,67) and induces severe respiratory distress and a persistence of fetal lung fluid postnatally (68). In the second step, sodium is actively pumped out of the cell into the interstitium through the basolateral membrane by the ouabain inhibitable Na+-K+-ATPase. Thus, Na+-K+-ATPase pump inhibition with ouabain consistently reduces liquid clearance in various species (62,66,69,70,71,72). To equilibrate the osmotic pressure, generated by the movement of Na+, water diffuses from the alveolar to the interstitial space either through specific water channels (aquaporins) or through the paracellular junctions (Fig. 17-2) (44,73,74). Although recent data demonstrate that aquaporin-4 messenger RNA (mRNA) in the perinatal epithelium is developmentally regulated and peaks at birth (74,75) there is no evidence that these channels regulate fluid clearance (Fig. 17-2) (10,44,55,72,73,76,77,78). The mechanisms of Na+ absorption through the pulmonary epithelium have been confirmed by isolation and culture of fetal alveolar type II cells (68,79,80,81,82).
The gene for the amiloride-sensitive epithelial sodium channel has been cloned; it consists of three homologous subunits called α-, β-, and γ-ENaC. The α subunit is a prerequisite unit for any channel activity. The expression of the three subunits is necessary for maximum ion transport (83). The α-ENaC (84) and the β- and γ-ENaC subunits (85) were detected in early midtrimester on the apical domain of human airway cells suggesting that sodium absorption might begin significantly before birth, even if secretion is still dominant. The three subunits increased sharply around the time of birth, were highest shortly after birth, and declined in parallel with endogenous plasma epinephrine concentration during the first week of life. The importance of the ENaC was confirmed by studies showing that α-ENaC knockout mice were unable to clear lung liquid from the alveolar spaces after birth resulting in death from respiratory failure (86). Mice with β- or γ-ENaC null mutations had delayed liquid clearance but had near-normal lung water content 12 hours after birth (86,87).
It is well known now that net alveolar fluid clearance occurs at a rapid rate late in gestation and that this clearance is driven by elevations of endogenous epinephrine. The critical link between β-adrenergic stimulation and lung fluid clearance was made in 1978 by Walters and Olver who found that intravenously infused epinephrine caused rapid absorption of lung fluid in near-term fetal lambs and that this response could be inhibited by prior treatment with propanolol (88). The intravenous epinephrine caused an
immediate and reversible increase in luminal electronegativity by stimulating the active transport of Na+ out of the lung lumen (55). In the absence of adrenergic stimulation the amiloride-sensitive Na+ channels remain closed (secretory state). Thus, the opened or closed state of the Na+ channels determines whether the fetal lungs, at any particular time, are secretory or reabsorptive (10). The epinephrine stimulation of amiloride-sensitive alveolar fluid clearance is mediated by cyclic adenosine monophosphate (cAMP) and Ca2+ likely acting as a intracellular second messenger (89,90).
immediate and reversible increase in luminal electronegativity by stimulating the active transport of Na+ out of the lung lumen (55). In the absence of adrenergic stimulation the amiloride-sensitive Na+ channels remain closed (secretory state). Thus, the opened or closed state of the Na+ channels determines whether the fetal lungs, at any particular time, are secretory or reabsorptive (10). The epinephrine stimulation of amiloride-sensitive alveolar fluid clearance is mediated by cyclic adenosine monophosphate (cAMP) and Ca2+ likely acting as a intracellular second messenger (89,90).
The induced reabsorption with epinephrine increases strikingly with advancing gestational age and can be induced by pretreatment of fetal sheep with the combination of corticosteroids and triiodothyronine (91). These two hormones are required to switch the effect of β-adrenergic stimulation from net chloride and liquid secretion to net sodium and liquid absorption (30,56,57,92,93). Consistent with in vivo observations, α-ENaC mRNA is increased by glucocorticoids in the primary cultures of alveolar type II cells FDLE, while the expression of β- and γ-ENaC mRNA is unaffected by glucocorticoid (94).
Another factor that appears to be particularly important in the switch of transepithelial liquid flow from secretion to absorption is the sharp increase in alveolar pressure of oxygen (PAO2) that occurs at birth. It was recently shown that fetal PO2 favored the development of fluid filled cyst-like structures while the rise of PO2 to postnatal levels reduced fluid in the cysts (95). The effect of oxygen seems to be the result of an increase in Na+-K+-ATPase activity and the response is also enhanced by glucocorticoids and thyroid hormones. Thus, at birth, epinephrine, oxygen, glucocorticoid and thyroid hormones interact to produce a permanent switch from secretion to absorption in the distal epithelium.
Although the switch from Cl– secretion to sodium absorption is the critical mechanism for lung fluid clearance at the time of delivery, other passive factors play a role in clearing the residual liquid present in potential air spaces at birth. The first is transient and peaks early and relates to enlarged transepithelial pores produced by spontaneous ventilation (22,29,47,51). Thus air inflation, by passive reabsorption down the transpulmonary pressure gradient associated with ventilation, shifts residual liquid from the lung lumen into the interstitium around distensible perivascular spaces of large pulmonary blood vessels and airways. These perivascular cuffs progressively diminish in size as the fluid is removed by small pulmonary blood vessels and lymphatics. Bland and associates (96,97) showed that the pulmonary circulation absorbs most of the residual liquid present in potential air spaces at birth and that elevated left atrial pressure or reduction of plasma protein concentration slows the rate of liquid clearance in mature animals. Although there is a small and transient increase in lymph flow after postnatal breathing begins, the amount of excess liquid drained postnatally by pulmonary lymphatics is only approximately 11% of the residual liquid in the lungs at birth (98). The second mechanism is the transvascular protein gradient that facilitates the movement of fluid from the essentially protein-free lung fluid into the interstitium, followed by passage of liquid into the bloodstream.
RESPIRATORY ADAPTATION
Fetal Breathing
The discovery of fetal breathing in the late 1960s immediately stimulated interest in the factors that control breathing in utero (99,100,101). Although of unknown purpose, because no gas exchange is involved, fetal breathing may represent preparation in utero for a vital function important in life. Shortly after its discovery, the Oxford group (102) showed that, although fetal breathing was influenced by fetal behavior, occurring essentially in rapid eye movement (REM) sleep, it was clearly regulated by other chemical factors, such as carbon dioxide and oxygen concentration. Subsequent work confirmed and expanded these findings by recording the electrical activity of the diaphragm and clearly demonstrating the central origin of the respiratory output in utero (103,104,105,106,107). Using ultrasonographic technology, breathing movements were also identified in the human fetus, being present approximately 40% of the time during late pregnancy, a figure similar to that in sheep (108,109,110,111).
The discovery of fetal breathing not only stimulated the development of the area of fetal assessment but it also brought a new dimension to the events occurring at birth. What has been traditionally called “the initiation of breathing at birth” must now be called “the establishment of continuous breathing at birth.” Breathing begins long before birth. The question is not what determines the appearance of breathing at birth, but what makes it continuous. From another angle, what makes fetal breathing episodic in late gestation and present only during low-voltage electrocortical activity? The answer to this question remains unknown.
Fetal breathing in sheep is mostly continuous in early gestation (90 to 115 days) but becomes episodic in late gestation, primarily occurring during periods of low-voltage electrocortical activity (99,101,107,112,113). During high-voltage electrocortical activity there is no established breathing present, but occasional breaths may surface after episodic, generalized, tonic muscular discharges associated with body movements (Fig. 17-3) (107). During low-voltage electrocortical activity, breathing is irregular, the diaphragmatic electromyelogram (EMG) being characterized by an abrupt beginning and end. The physiologic mechanism responsible for the occurrence of fetal breathing only during low-voltage electrocortical activity is unknown.
Modulation of Fetal Breathing by CO2, O2, and Neurochemicals
Many studies clearly show that the fetal breathing apparatus is capable of responding well to chemical stimuli and other agents known to modify breathing postnatally. Thus it
became clear that the fetus responds to an increase in arterial carbon dioxide pressure (PaCO2) with an increase in breathing (102,108,114,115,116,117,118). The increased breathing activity is prolonged into the transitional high voltage electrocochleography but does not continue into the established (Fig. 17-4) (118). There is much evidence suggesting that the actions of CO2 are central.
became clear that the fetus responds to an increase in arterial carbon dioxide pressure (PaCO2) with an increase in breathing (102,108,114,115,116,117,118). The increased breathing activity is prolonged into the transitional high voltage electrocochleography but does not continue into the established (Fig. 17-4) (118). There is much evidence suggesting that the actions of CO2 are central.
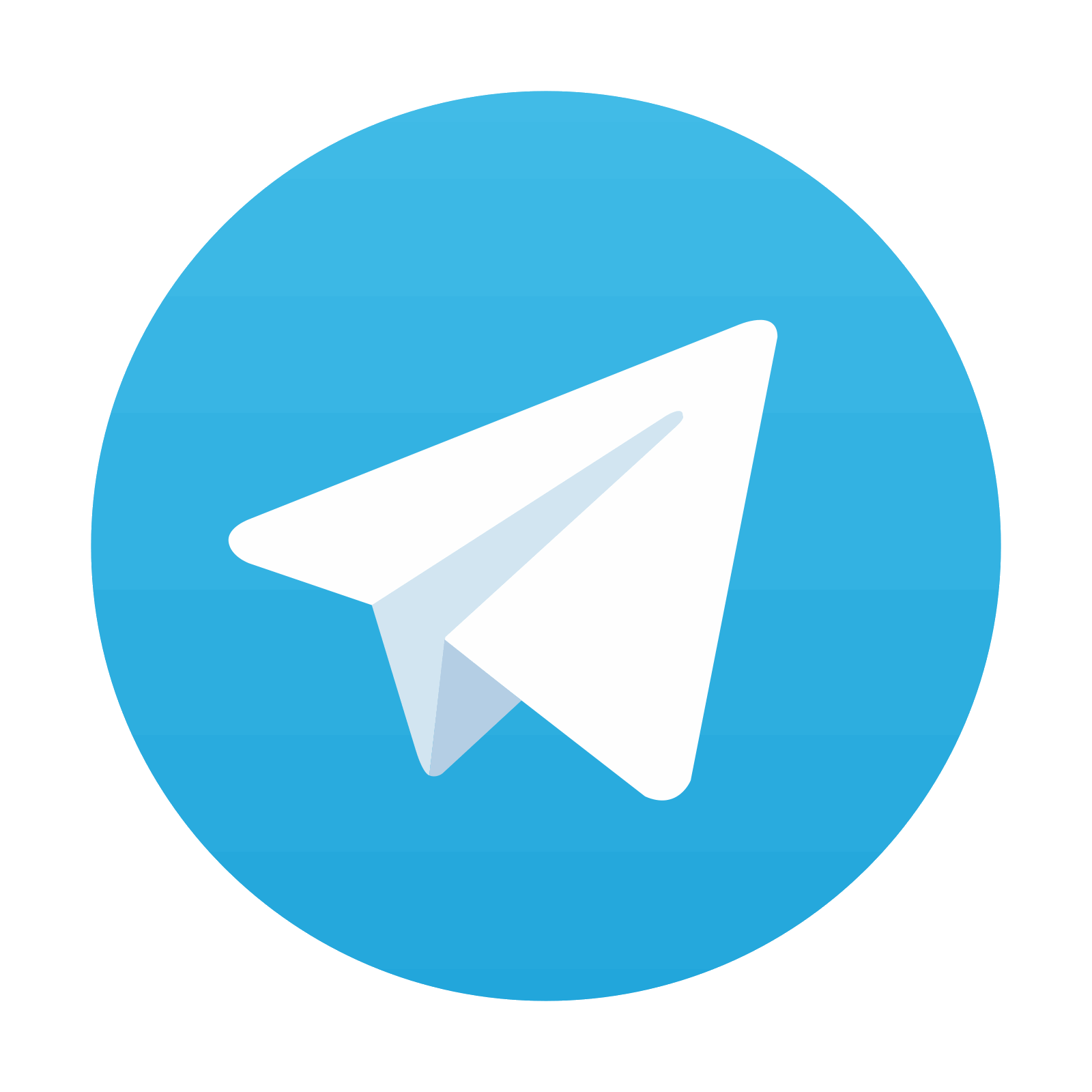
Stay updated, free articles. Join our Telegram channel
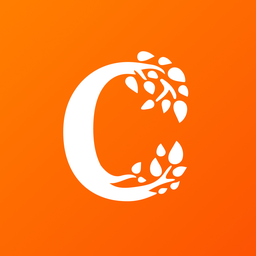
Full access? Get Clinical Tree
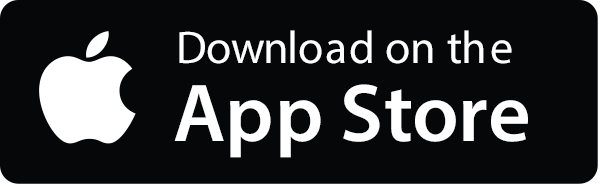
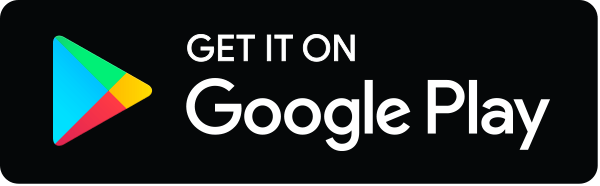