The effect of changes in intrathoracic pressure on cardiac function
Spontaneous ventilation and normal heart
Positive intrathoracic pressure and systemic venous return
The effects of changes in intrathoracic pressure on pulmonary blood flow
The effect of changes in intrathoracic pressure on left heart function
The effects of positive end-expiratory pressure on cardiovascular function
Positive intrathoracic pressure in lung disease: the acute respiratory distress syndrome
The effect of changes in intrathoracic pressure in congenital heart disease
Intrathoracic pressure in tetralogy of Fallot and Fontan physiology
Positive pressure ventilation and the bidirectional cavopulmonary shunt
Positive pressure ventilation and ventricle lesions with duct-dependent systemic perfusion or systemic to pulmonary artery shunts
Positive pressure ventilation in left to right intracardiac shunts
Cardiopulmonary interactions in acquired heart disease
Positive pressure ventilation and heart failure
The use of noninvasive continuous positive airway pressure in heart failure
53.2 The Effect of Changes in Intrathoracic Pressure on Cardiac Function
53.2.1 Spontaneous Ventilation and Normal Heart
Perhaps the easiest way to begin to understand the fundamentals of the complex interaction between the systemic and pulmonary circulations within the thorax is to use a model of two pumps connected in series enclosed within a chamber where the pressure is constantly changing. The reservoir for the filling of the right heart lies partly outside the thorax and is consequently subject to atmospheric or intra-abdominal pressure (IVC), whereas some of the large venous connections (SVC) are intrathoracic and subject to pleural pressure (Fig. 53.1). On the other hand, the reservoir for left heart filling (the pulmonary circulation) and the systemic pumping chamber lie entirely within the thorax, although the pump ejects against a high impedance which is largely extrathoracic (systemic vascular resistance). Since pleural pressure is constantly changing during the respiratory cycle, it follows that the resulting fluctuations in intrathoracic pressure will affect the output from the pump by altering preload or filling on the right side and afterload or ejection on the left side.
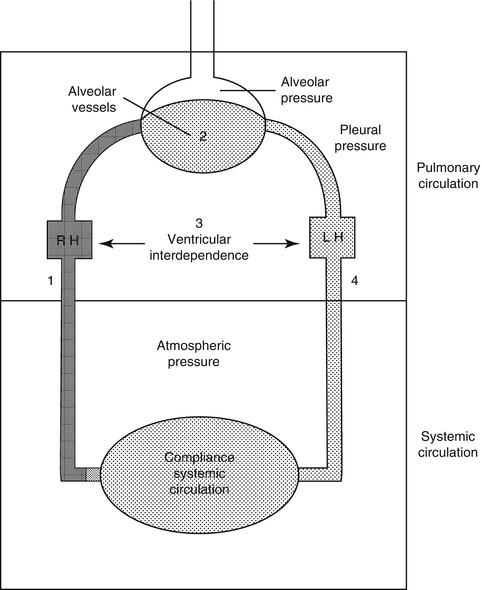
Fig. 53.1
A summary of cardiopulmonary interactions in a two-compartment model of the circulation. Both the right and left heart are subjected to pleural (intrathoracic) pressure, while the systemic vasculature is subjected to atmospheric or, in the case of the IVC, intra-abdominal pressure (From Permutt (1989))
The forces that govern venous return and how these are influenced by spontaneous respiration have been defined in the classic experiments of Guyton et al. (1954, 1955, 1957, 1958). The pressure generated for return of blood flow to the heart is the driving pressure (mean systemic pressure) minus the downstream pressure opposing venous return which in this instance is the right atrial pressure (Pra). Acute elevations in right atrial pressure will result in a fall in cardiac output until compensated for by a change in compliance in the venous capacitance system. The pressure within the venous system is determined by the compliance and the volume of blood in the vascular bed and, with total circulatory arrest, the mean systemic pressure is 7 mmHg on both sides of the heart (Guyton et al. 1955). The negative pleural pressure that occurs during a normal spontaneous inspiration produces a fall in right atrial pressure and hence the upstream pressure the venous capacitance sees, resulting in increased atrial filling (Fig. 53.2). The filling pressure gradient for venous return to the right atrium is usually around 5 mmHg and is determined by the difference between the extrathoracic and intrathoracic venous pressures. As negative intrathoracic pressure increases the caliber of intrathoracic veins, one might suppose that a dramatic increase in venous return might occur during a reduction in right atrial pressure produced by a deep inspiration. In the normal human, there is flow limitation as this augmentation of venous return by negative intrathoracic pressure is limited by the fact that when pleural pressure becomes subatmospheric, the extrathoracic veins collapse at the thoracic inlet, limiting flow, while the intrathoracic cavae are maintained patent by a series of valves.
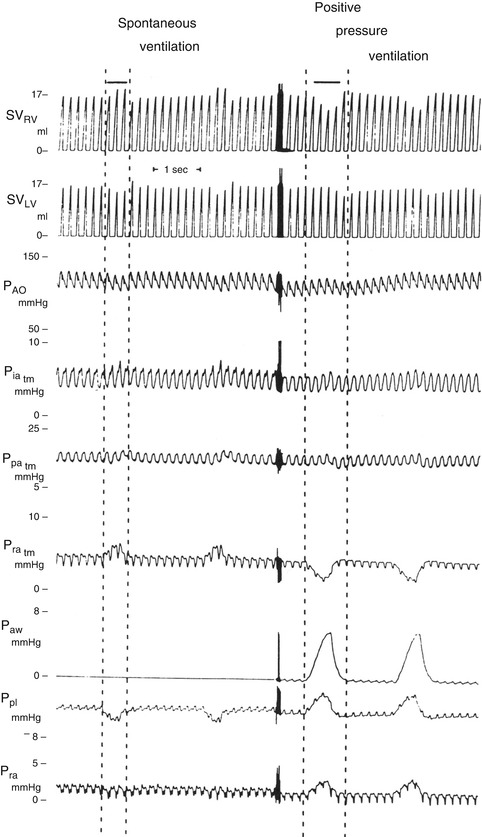
Fig. 53.2
Changes in hemodynamics associated with spontaneous and positive pressure ventilation in the normal human. The area between the dashed lines represents the inspiratory phase. The reduction in pleural pressure during spontaneous inspiration increases right atrial filling and the right atrial pressure rises together with right ventricular stroke volume. There is a simultaneous fall in left ventricular stroke volume. During positive pressure ventilation right heart filling decreases, while LV stroke volume rises. For a more detailed explanation see text (From Pinsky (1991))
The interaction between respiratory and cardiac function is a complex one with major differences occurring under conditions of spontaneous or positive pressure respiration. These are illustrated in Fig. 53.2 which shows hemodynamic pressure changes during ventilation. The dashed lines represent the inspiratory phase of the respiratory cycle. During spontaneous breathing pleural pressure becomes negative during inspiration increasing the pressure gradient for venous return, transmural right atrial pressure (Pratm) rises and right ventricular stroke volume (SVRV) increases. Coincident with this there is a transient fall in left ventricular stroke volume (SVLV) which is then augmented within a couple of cardiac cycles. The reasons suggested for this include the pooling of blood in the pulmonary circulation due to lung expansion, right heart filling causing a change in left ventricular diastolic compliance, or increased afterload on the left ventricle due to negative intrathoracic pressure. Positive inspiratory pressure on the other hand leads to a fall in Pratm as the rise in intrathoracic pressure decreases the gradient for venous return, filling of the atrium is impeded, and SVRV falls, but there is a phase lag before this reduction is seen in the left heart. As intrathoracic pressure increases there is a very transient rise in SVLV due to either reduced afterload on the LV or enhanced flow from pulmonary capillaries to the LA associated with the increase in intrathoracic pressure. This is more than offset by the subsequent fall in SVLV as right-sided events become predominant.
Two further factors influence the performance of the left and right ventricles; because there are cardiac muscle fibers that interconnect both ventricles and they share a common septum, changes in the contractile state of one ventricle will be reflected in the other, a phenomenon known as ventricular interdependence (Bove and Santamore 1981; Jardin 2003; Lloyd 1982). Both pumping chambers are also constrained within a viscoelastic membrane (the pericardium) which, while it does not normally have a significant influence on function, may inhibit contraction in situations where intrapericardial pressure increases. The interaction between respiratory and cardiac function is a complex one with major differences occurring under conditions of spontaneous or positive pressure respiration. Finally changes in lung volume, independent of any change in intrathoracic pressure, alter the caliber of the pulmonary vascular bed and thereby influence right ventricular afterload.
During ventilation gas moves in and out of the lung under the influence of changes in intrathoracic pressure (ITP). This results in important changes in hemodynamics which differ according to whether the change in ITP is negative or positive during inspiration or alveolar pressure is zero or positive at end expiration (PEEP). These changes are also influenced by underlying cardiac or respiratory disease. Although the term ITP is used generically to cover all pressure changes within the mediastinum, sometimes a differentiation needs to be made between it, pleural, alveolar, and transpulmonary (alveolar minus pleural) pressure.
53.2.2 Positive Intrathoracic Pressure and Systemic Venous Return
With the introduction of positive pressure ventilation, it was recognized from the outset that there were important effects on hemodynamics which were entirely different from spontaneous respiration. Cournand et al. (1948) was the first to show the association between the decreased cardiac output and increased airway pressure in normal humans with mask positive pressure ventilation (PPV) experiments over 50 years ago. The explanation for this finding was that increased intrathoracic pressure is transmitted to the systemic venous system which resulted in reduced caval blood flow. The rise in right atrial pressure reduced the gradient for right atrial filling, which in turn leads to fall in right ventricular stroke volume (Fig. 53.2). The finding has been confirmed in numerous studies since then which have shown that in the absence of cardiopulmonary disease, positive intrathoracic pressure will reduce cardiac output through the mechanism of decreased venous return (Brecher and Hubay 1955; Charlier et al. 1974; Pinsky et al. 1985; Scharf et al. 1977, 1980) especially in situations of absolute or relative hypovolemia, while in the hypervolemic state pulmonary venous return to the LV would be increased due to augmented antegrade flow. The most graphic demonstration of this effect is when the increase in pleural pressure occurs without a change in lung volume as in a Valsalva maneuver (Brooker et al. 1974; Korner et al. 1976; Parisi et al. 1976).
What has been less widely appreciated is that in the normal situation there is an initial increase in both aortic pressure and left ventricular output early in the inspiratory phase of positive pressure ventilation before falling towards the end of inspiration (Scharf et al. 1980; Jardin 2004; Morgan et al. 1966, 1969) and that this increase in aortic pressure is more pronounced at faster respiratory rates (Fig. 53.3). In contrast, changes in respiratory rate have little effect on pulmonary artery flow, which declines during inspiration and rises during expiration. It therefore appears that increased pleural pressure will have different effects on the right and left heart output at different phases of the respiratory cycle where a fall in pulmonary artery flow and rise in pulmonary artery pressure are seen at the same time as there is a rise in both pressure and flow in the aorta early in the inspiratory phase of positive pressure ventilation. These differing effects require more detailed analysis. Among the theories that have been advanced to account for these differences are a phase lag due to pulmonary blood transit time, reduction in left ventricular afterload associated with positive pleural pressure, increased left ventricular preload due to forward flow of pulmonary venous blood due to lung expansion, and increased left ventricular compliance due to ventricular interdependence. The fact that pulmonary transit time has little or no bearing on the asynchrony between the right and left heart has been demonstrated in the intact animal by Robotham et al. (1983). In these experiments right heart output replaced by a roller pump to maintain a constant left-sided preload, aortic flow still increased during the early phase of inspiration despite the elimination of the variation in right ventricular output. In a further series of experiments in open-chest animals, where pleural pressure is no longer a factor and with the right heart decompressed eliminating the ventricular interdependence factor, Robotham has also been able to show that the application of positive pressure to the lung still resulted in increased left ventricular output (Robotham and Mintzner 1979).
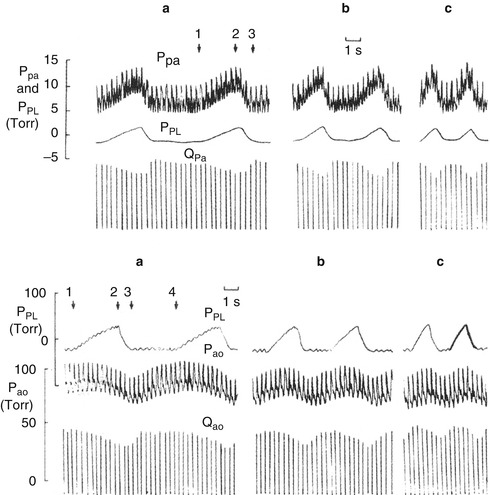
Fig. 53.3
Pulmonary and aortic blood flow during increasing rates of positive pressure ventilation (a–c) measured at 1) beginning of inspiration, 2) end inspiration, 3) end expiration, and 4) beginning of next inspiration. Aortic flow (Q ao) begins to decrease shortly after the start of each inspiration reaching a nadir at end expiration. Aortic pressure (P ao) on the other hand rises slightly to a peak which occurs closer to end inspiration at more rapid respiratory rates and reaches a nadir which is almost simultaneous with the nadir in Q ao (From Scharf et al. (1980))
Many of the studies of the effect of positive pressure ventilation on the right heart have been done in steady-state conditions after increases in ITP rather than examining the instantaneous effects of increases in airway pressure (Pinsky 1984). Also, many of these studies did not take into account the potential impact of the changes in intravascular volume status that might occur in patients might have on the relationship between ITP and venous return. Important information on caval blood flow is now available using echocardiography and pulsed Doppler techniques. Studies on changes in ITP in normal animals who are not hypovolemic have shown that mean systemic pressure is maintained even as ITP increases (Fessler et al. 1988). The explanation for this is as the diaphragm descends during inspiration, the increase in intra-abdominal pressure results in increased venous return by enhancing the splanchnic IVC flow (Takata and Robotham 1992; Takata et al. 1990). Under relatively hypervolemic conditions. Pra exceeds intra-abdominal pressure (Pab), analogous to zone III conditions described for pulmonary blood flow in the lung (West et al. 1964). In situations of hypovolemia, however, Pab exceeds Pra (zone II) and the total IVC venous return is reduced (Takata and Robotham 1992; Kitano et al. 1999). These findings have been confirmed in humans. Van den Berg et al. (2002) has shown that in fluid-resuscitated postoperative cardiac surgical patients, increases of airway pressure up to 20 cmH2O resulted in no change in cardiac output or RV end-diastolic volume. This was ascribed to the fact that there were parallel increases in Pra as well as Pab and therefore there was no effect on venous return. This study demonstrates that venous return can be maintained when ITP and Pra increase as long as mean systemic pressure increases by an equivalent amount. This happens because the rise in Pab compresses the liver and squeezes the lungs. The same does not apply if the patient is hypovolemic when increased ITP results in zone 2 conditions and collapse of the thoracic portion of the vena cava. A summary of these studies would conclude that venous return and stroke volume of the right heart increase due to the negative intrathoracic pressure associated spontaneous breathing, while positive ITP only significantly impacts on right heart filling when patients are hypovolemic or the level mean airway pressure is so high as to render them relatively hypovolemic. This will be discussed further in the section on ARDS. Paradoxically, in situations where severe right ventricular failure results in profoundly low cardiac output, ventilation with high peak inflation pressures at rapid rates may result in a rapid improvement in hemodynamics. Serra et al. (1988) have reported a dramatic improvement in four children with profound right ventricular failure after corrective cardiac surgery when switching from conventional ventilator settings to higher-frequency (50/min) high-volume (TV 30 ml/kg) ventilation. The increased MAP in this situation probably acts as a right ventricular assist device in increasing forward flow from a dilated and noncontractile right ventricle in much the same way that it produces forward flow in simultaneous ventilation-compression CPR.
Important new insights into the effect of positive intrathoracic pressure on right heart function in critically ill patients have been gained from the use of echocardiography and pulsed Doppler. These show that when tidal volume is progressively increased, the RV has to generate an increasingly higher pressure to open the pulmonary valve (Jardin et al. 1989). Increases in transpulmonary pressure (airway pressure minus pleural pressure) and tidal volume during the inspiratory phase of positive pressure ventilation result in a sharp but transient reduction in flow acceleration in the pulmonary artery. However, when airway pressure was increased without a change in tidal volume, there was no effect on RV afterload.
53.2.3 The Effects of Changes in Intrathoracic Pressure on Pulmonary Blood Flow
Ventilation influences PVR in a number of different ways. Changes in airway pressure (Paw) transmitted to the alveoli during spontaneous and positive pressure ventilation alter pulmonary blood flow by changes in the caliber of pulmonary capillaries. Lung distention per se has significant neurohormonal effects on PVR and right ventricular function via the vagus nerve and the release of prostanoids, ADH, atrial natriuretic peptide, and catecholamines (Berry et al. 1971; Edmonds et al. 1969; Farge et al. 1995; Frass et al. 1993; Glick et al. 1969; Payen et al. 1987). There is also the important effect of pH on PVR mediated by altering PaCO2, independent of any change in lung volume.
For the purposes of examining the effect of the change in Paw on pulmonary vascular resistance, it has been convenient to think of pulmonary vessels as being divided into two functional groups; the large pulmonary vessels and the heart which sense changes in interstitial pressure in the lung and those which are exposed to an extravascular pressure which reflects alveolar pressure (intra-alveolar vessels). The change in blood flow in the intra-alveolar vessels depends on their position within the different zones of the lung as outlined by Permutt and West (West et al. 1964; Permutt et al. 1962) who defined the relationship between pressures in the alveolus (PA) and pulmonary artery (Pa) and left atrium (Pla). Where zone I (lung apex) conditions apply, the pressure within the arterial end of the vessel is less than alveolar pressure (PA > Pa > Pla) and is therefore insufficient to open the vessels, which remain collapsed and there is no flow (Hughes et al. 1968). These conditions exist in the uppermost parts of the lung in the upright human or in the superior part of the lung when lying supine. Where zone II conditions apply (Pa > PA > Pla), arterial pressure is higher than alveolar and the intra-alveolar vessels behave like Starling resistors surrounded by alveolar pressure where flow depends on the difference between arterial and alveolar pressures and is independent of changes in left atrial pressure. These conditions predominate in the mid zone of the lung in the upright and supine human and can also be seen during the inspiratory phase of positive pressure ventilation (Jardin and Vieillard-Baron 2003). In zone II conditions, the back pressure to right ventricular ejection is alveolar rather than left atrial pressure, and the relevant resistance is only that between the pulmonary artery and the downstream end of the alveolar vessels. An increase in lung volume produces an increase in back pressure to right ventricular ejection compared with the pressure around the heart and increased afterload. This requires that an approximately equal increase in pressure be produced in the pulmonary artery and alveolus to maintain pulmonary blood flow, which translates into increased right ventricular wall stress. Thus, an increase in alveolar relative to pleural pressure increases right ventricular afterload, and it is this change in alveolar pressure relative to arterial that can produce a marked degree of increased afterload seen in acute asthma (Permutt 1973). In zone III conditions (Pa > Pla > PA), the pressure in the venous side of the capillary is higher than alveolar pressure, and pulmonary blood flow behaves like a Starling resistor where flow is independent of alveolar pressure and is governed by the difference between pulmonary arterial and venous pressures. These conditions predominate in the dependent lung regions.
The effect of a change in lung volume on extra-alveolar vessels is somewhat different. An increase in lung volume during inspiration increases radial traction on extra-alveolar vessels, increasing their caliber and causing a fall in pulmonary vascular resistance and a decrease in right ventricular afterload. The net effect of these various changes is that pulmonary vascular resistance is lowest at FRC and is minimally changed at airway pressures of 5–10 cmH2O and rises in situations where vessels are compressed during lung collapse or where there is overdistention of the lung. Loss of FRC, as seen with the development of pulmonary edema or atelectasis, will result in a rise in pulmonary vascular resistance, as will overdistention of the lung due to airway obstruction or high peak airway pressure ventilation that puts the lung on the flat portion of the pressure-volume curve. In this situation zone I conditions would predominate throughout the lung (PA > Pa > Pla) and pulmonary vascular resistance would be increased.
53.2.4 The Effect of Changes in Intrathoracic Pressure on Left Heart Function
The changes in intrathoracic pressure produced by respiration have important and hitherto underappreciated effects on left ventricular function which assume greater importance in the failing heart. In order to evaluate the significance of these various forces and the effect of positive or negative intrathoracic pressure on left ventricular preload and afterload, it is important to understand the changes in cardiac output and left heart function secondary to respiration.
The most commonly observed change in left heart function that occurs with spontaneous respiration is an initial fall in arterial pressure during inspiration due to a decrease in left ventricular stroke volume (Fig. 53.2). The reasons for this have been largely attributed to events occurring on the right side of the circulation and include (1) the pooling of blood in the pulmonary circulation due to lung expansion, (2) a phase lag between right and left ventricular output, (3) the stimulation of systemic baroreceptors or pulmonary stretch receptors, and (4) impeded right heart filling causing a change in left ventricular diastolic compliance or ejection mediated through ventricular interdependence. Although studies of pulmonary transit time have shown that it takes one to two cardiac cycles for a change in right-sided output to be reflected in the left side (Franklin et al. 1962; Maloney et al. 1968), the phase lag theory is unlikely to fully explain the decrease in left ventricular ejection associated with a reduction in intrathoracic pressure. Changes in right heart output must also affect the left side as the two circulations are connected in series, which accounts for the observation that blood pressure and left ventricular stroke volume may rise after an initial fall. In this situation, the increase in venous return eventually overrides the other factors that tend to impede left ventricular output. Neural receptors that have been suggested to influence left ventricular function include stretch receptors in the lung mediated via the vagus nerve and intra- and extrathoracic baroreceptors that are mediated by the autonomic system. There is reliable experimental data to suggest that neither of these is likely to be a major mechanism in the fall of left-sided output. Robotham et al. (1979) found that there were still significant falls in left ventricular stroke volume in vagotomized animals during a Mueller maneuver (inspiratory effort against a closed glottis), where intrapleural pressure falls but lung volume remains unchanged. A decrease in left ventricular stroke volume during inspiration is still seen even after autonomic blockade of vagal and sympathetic efferent nerves. If left atrial and ventricular diastolic pressures were measured relative to atmosphere, then one could demonstrate a fall in these pressures during inspiration, which would support the concept that the principal cause of the decrease in left-sided output during inspiration was pooling of blood in the lungs secondary to lung expansion. However, it has been shown that during inspiration in both the intact animal and the isolated lung preparation, pulmonary venous return actually increases at the same time that left ventricular stroke volume is falling (Guntheroth et al. 1967; Howell et al. 1961). This apparent paradox is explained when these pressures are related to intrapleural pressure where it can be shown that transmural (intracavity minus intrapleural) filling pressures on the left side actually increase during inspiration. Thus it is more valid to define the afterload as being the left ventricular transmural pressure which in this instance is the intracavity pressure minus the intrapleural pressure (Fig. 53.4).
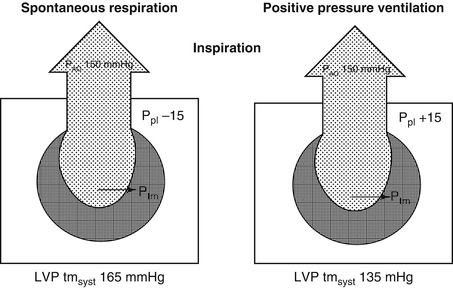
Fig. 53.4
Changes in afterload on the left ventricle associated with the inspiratory and expiratory phase of spontaneous ventilation. The numbers for pleural and intracavity pressure are arbitrary and chosen only to illustrate the point that afterload (end-systolic pressure) increases during inspiration. LVtm syst left ventricular transmural systolic pressure
The observation that afterload increases with negative intrathoracic pressure has been confirmed by studies of left ventricular function during spontaneous breathing with increased inspiratory loads which demonstrated that both left ventricular end-diastolic and end-systolic volumes are increased (Scharf et al. 1979a, b). However this occurs independently of changes in lung volume as has been demonstrated in experiments which showed that afterload was found to increase during a Mueller maneuver. Both Brinker et al. (1980) and Guzman et al. (1981) have also confirmed septal wall displacement during the Mueller maneuver which was associated with decreased diastolic compliance and volume of the left ventricle. Sustained decreases in intrathoracic pressure have also been shown clinically to result in mild degrees of left ventricular dysfunction when associated with ischemic heart disease (Scharf et al. 1981).
Various mechanisms have been invoked to explain the marked fall in left ventricular stroke volume arterial blood pressure that occurs with large negative intrathoracic pressures during spontaneous respiration during severe airway obstruction. It has been difficult to determine whether this is due to decreased left ventricular filling or increased afterload because left ventricular filling and emptying may occur simultaneously with inspiration extending over several cardiac cycles. In a series of experiments, Peters et al. (1988a, b) has attempted to clarify this issue by synchronizing negative intrathoracic pressure with systole and diastole independently. The findings would suggest that negative intrathoracic pressure with the airway obstructed during systole reduces left ventricular stroke volume predominantly by increasing afterload and impedance to blood flow out of the thorax. When negative intrathoracic pressure was synchronized with diastole, left ventricular output fell due to ventricular interdependence.
Since the generation of large negative intrathoracic pressures can impede both diastolic and systolic performance of the left ventricle, it is not surprising that this will occasionally result in acute left ventricular failure and the development of pulmonary edema. This has been well documented during acute upper airway obstruction occurring with laryngospasm during anesthesia (Cozanitis et al. 1982; Jackson et al. 1980; Lee and Downes 1983) and with croup and epiglottitis (Oswalt et al. 1977; Stradling and Bolton 1982; Travis et al. 1977). It may also occur following the relief of upper airway obstruction (Sofer et al. 1984) and during status asthmaticus where at the peak of inspiration negative intrathoracic pressures of up to minus 40 cmH2O can be produced (Stalcup and Mellins 1977). The overall effect of a negative intrathoracic pressure on left ventricular function is therefore a balance between its effect on the preload and left ventricular systolic ejection (afterload). The increase in afterload due to negative pleural pressure is one of the mechanisms responsible for the worsening of heart failure seen with obstructive sleep apnea (Naughton et al. 1994, 1995a). It may become even more pronounced in situations of decreased lung compliance such as pulmonary edema or lung disease where there are increasingly negative swings in intrathoracic pressure (Naughton et al. 1995b).
The effect of positive pressure ventilation on the left heart is particularly relevant in critical care medicine. The following considerations need to be taken into account: (a) right heart preload and septal shift influence LV end-diastolic volume; (b) there is a phase lag before this is manifest in left heart output; (c) the effect is most pronounced at end inspiration; (d) there is a difference between increased pleural pressure, which impedes venous return, and increased lung volume, which both compresses the heart and increases the pulmonary extra-alveolar capacity; and (e) at peak inspiration ITP affects systolic performance and this is significantly influenced by the underlying function of the LV. Attempts have been made to separate these different effects by examining ventricular function during apnea, by synchronizing ventilation with ventricular systole using a jet ventilator, by changing alveolar pressure independent of ITP with an abdominal binder, and by examining the effect of PPV in a heart failure model induced by beta blockade.
Positive pressure ventilation alters left ventricular function by both mechanical forces and changes in lung volume. An increase in ITP alters diastolic compliance and pericardial pressure. The net result is a decrease in left ventricular end-diastolic volume due to decreased preload but no change in systolic right ventricular function. Several studies have shown that left ventricular dimensions are altered by both intermittent positive pressure ventilation and PEEP in that the septal to free wall and anterior to posterior minor axis dimensions diminish, which is consistent with an overall decrease in left ventricular end-diastolic volume (Robotham et al. 1979, 1985; Visner et al. 1983). The effect is greater in the septal to free wall dimension, which suggests that ventricular interdependence is the important mechanism that contributes to the decreased left ventricular preload seen with the application of PEEP.
Positive pressure ventilation-induced changes in lung volume also affect left ventricular function independent of the change in intrathoracic pressure. Lung expansion changes the capacitance of the pulmonary venous system thereby altering pulmonary blood flow depending on the underlying pulmonary blood volume and vascular tone (Brower et al. 1985). Increasing lung volume will also restrict cardiac filling in a similar fashion to cardiac tamponade by encroachment of the lung on the cardiac fossa (Lloyd 1982). This may have important implications in positive pressure ventilation in status asthmaticus where overinflation of the lung caused by rapid respiratory rates and inadequate expiratory time can result in low output and cardiac arrest. A dramatic illustration of this is provided in a case report of an adult with severe asthma who had a cardiac arrest after intubation and had all the features of cardiac tamponade (Rosengarten et al. 1991). The usual resuscitation techniques failed including prolonged expiratory time ventilation. After CPR was abandoned spontaneous circulation resumed after 3 min of apnea. This demonstrates how severe gas trapping can have a profound on cardiac function.
Recently more sophisticated techniques for measuring left heart performance using cardiac echo and conductance catheters have become available. These have allowed investigators to measure chamber size and display pressure-volume loops of the LV while lung volume and ITP were altered (Denault et al. 2001). These studies have shown that the influence of ventilation on LV function is complex and influenced by pulmonary mechanics, circulating volume status, and the underlying contractile state of the ventricle. When ventricular function is normal and there is a normal circulating volume, the preload effect predominates, and increase in ITP at end inspiration results in a decrease in LV end-diastolic volume, without a change in systolic performance. As this occurs at the same time as there is a rise in LV end-diastolic pressure, the purported mechanism is compression of the heart by the lungs. When contractility is impaired and ventricular volumes are increased, positive pressure inspiration reduces LV end-systolic volume by lowering afterload and improving ejection (Denault et al. 2001). This has important implications for the treatment of heart failure, as will be discussed later.
53.2.5 The Effects of Positive End-Expiratory Pressure on Cardiovascular Function
The effects of PEEP on cardiovascular function have been a source of major interest in critical care medicine since the original description of its use in ARDS over 30 years ago. These include the potential for diminished myocardial contractility and ventricular compression by lung distention (Lloyd 1982) as well as the overall effect on cardiac output. However, it is frequently difficult to separate the effect of PEEP independent of the peak airway pressure as a change in both results in a change in the mean airway pressure (MAP). The application of PEEP in the normal human and animal heart results in a fall in cardiac output due to decreased preload (venous return) and increased afterload (pulmonary vascular resistance) (Rankin et al. 1982). In addition, Doppler flow studies have shown backward flow of blood through the tricuspid valve (Jullien et al. 1995). PEEP, in addition to increasing pleural pressure, will increase lung volume and FRC depending on lung and chest wall compliance. If it overdistends the lung and increases pulmonary vascular resistance, an increase in right ventricular volume will occur which may adversely affect left ventricular compliance by leftward shift of the intraventricular septum. However, in the situation where the appropriate amount of PEEP is being used, lung volume will be recruited and the end-expiratory volume will be at funtional residual capacity (FRC) and pulmonary vascular resistance will fall. High levels of PEEP have been shown to compromise flow in a marginal right coronary circulation because of decrease in flow associated with the rise in right ventricular systolic pressure and intrapericardial pressure (Fessler et al. 1988; Bishop et al. 1976; Brooks et al. 1971). Similar observations on the differing effect of PEEP on right ventricular ejection fraction have been made on adults with ischemic heart disease after cardiopulmonary bypass (Boldt et al. 1988). Patients with pronounced right coronary artery stenosis had diminished right ventricular ejection fraction and increased right ventricular end-diastolic volume, whereas there was no effect in patients with minor coronary artery stenosis.
53.3 Positive Intrathoracic Pressure in Lung Disease: The Acute Respiratory Distress Syndrome
One of the most important areas where positive intrathoracic pressure potentially impacts on cardiovascular function is in patients with acute respiratory distress syndrome (ARDS). The current focus on lung recruitment strategies using high PEEP as part of the open lung approach, while improving oxygenation, pays scant attention to what impact this might have on cardiac function. If the only consideration were an improvement in oxygenation, then it would simply be a case of increasing PEEP until the best PaO2 at the lowest FiO2 was achieved. However, the potential adverse effects of PEEP on cardiovascular function mean that the most important therapeutic goal is the level of PEEP that gives the best combination of oxygenation and cardiac output, thereby achieving maximum oxygen delivery (oxygen content x cardiac output). Given that ARDS is a multisystem disease, it is important that we focus our attention on how positive pressure ventilation, PEEP, and lung disease affect the other major organ that shares the chest cavity.
In the past, attempts to define the effects of PEEP on the cardiovascular system have relied on studies in intact animals and small studies in humans using intracardiac pressure measurements from pulmonary artery catheters in normal and disease states. These studies have shown that the magnitude of changes depends partly on factors that influence the underlying cardiovascular status (circulating volume, ventricular dysfunction, increased pulmonary vascular resistance) and on factors within the lung that may modulate the transmission of airway (alveolar) pressure to the pleural space and consequently to the heart and vascular structures. Decreased lung compliance will reduce pressure transmission while increased thoracic compliance will enhance it (Jardin et al. 1985a; Pontoppidan et al. 1977). Taking all these factors into consideration, it is not unusual to see a fall in cardiac output and stroke volume with high levels of positive pressure ventilation with PEEP in ARDS. The mechanisms that have been invoked as a cause for this decrease include decreased venous return, increased right ventricular afterload, decreased left ventricular compliance, and decreased ventricular contractility. However, these studies are limited by the fact that pressure was measured rather than changes in volume.
The importance of right ventricular function in patients with ARDS has been the subject of increasing attention (Jardin et al. 1985b, c, 1989; Jardin and Vieillard-Baron 2003; Hurford and Zapol 1988; Jardin and Bourdarias 1997; Vieillard-Baron and Jardin 2003; Vieillard-Baron et al. 2001a). As is seen with positive pressure ventilation in the normal lung, the increase in pleural pressure with PEEP will reduce venous return to the right heart in ARDS (Dhainaut et al. 1986; Potkin et al. 1987; Viquerat et al. 1983), and this is frequently compensated for by giving fluid to increase filling pressures. In patients with severe acute respiratory failure, levels of PEEP above 10 cmH2O have also been associated with an increase in right ventricular afterload (Dhainaut et al. 1986; Viquerat et al. 1983; Calvin et al. 1981; Jardin et al. 1984). In the setting of severely depressed baseline right ventricular ejection in humans, the application of PEEP has been shown to result in the depression of contractile function (Schulman et al. 1988). Following right coronary artery ligation in animals, right ventricular ejection fraction declined associated with an increase in end-systolic volume (Schulman et al. 1990). Even in the absence of overt ischemia, acute right heart dysfunction has been identified as a cause of morbidity and mortality in ARDS (Monchi et al. 1998). This is not only due to the effects of ventilation and PEEP but also as a result of the underlying lung disease. The alveolar collapse and microvascular obstruction cause an increase in PVR and pulmonary artery pressure (Zapol and Snider 1977). There is an association between this increase in PVR and mortality (Squara et al. 1998).
The more widespread use of echocardiography and pulsed Doppler has improved our understanding of the effect of PEEP on right heart function or cor pulmonale in ARDS. Before the advent of reduced tidal volume ventilation, a 61 % incidence of acute cor pulmonale, defined as paradoxical septal motion with acute RV enlargement, was reported in adults with ARDS (Fig. 53.5) (Jardin et al. 1985c). More recently the same investigators have reported a 25 % incidence in a study where tidal volume was limited to 8 ml/kg (Vieillard-Baron et al. 2001a). Another study compared the effect on pulmonary blood flow at zero PEEP with two other levels, one determined by the highest value of lung compliance and the second the coordinates for the lower inflection point on the lung pressure-volume curve (Schmitt et al. 2001). They found that the PEEP level that had the least effect on RV impedance was that associated with the highest compliance (6 ± 3 cmH2O), a number remarkably similar to that found in the best PEEP study by Suter 35 years ago (8 cmH2O) (Suter et al. 1975). In a landmark study in 1981, Jardin et al. (1981) measured right and left heart pressures together with measurements of chamber size with echo while PEEP levels of up to 25 cmH2O were applied. He found that increasing levels of PEEP were associated with a gradual decrease in left ventricular end-systolic and end-diastolic dimensions as lung hyperinflation induced increased RV afterload as leftward shift of the septum encroached on the LV cavity (Fig. 53.6). The thin-walled RV dilates as afterload increases, but the free wall is constrained by the pericardium, and therefore it can only dilate leftwards as the end-diastolic volume increases. In previous studies the reduction in cardiac output was frequently compensated for by expansion of circulating volume, which increased right ventricular preload and right ventricular myocardial segment length (Dhainaut et al. 1986; Viquerat et al. 1983; Calvin et al. 1981; Jardin et al. 1981; Prewitt et al. 1981; Qvist et al. 1975). In these studies, however, the fall in cardiac output could be compensated for by infusing volume only in patients who were on low levels of PEEP. Left ventricular contractility was unaltered by any level of PEEP.
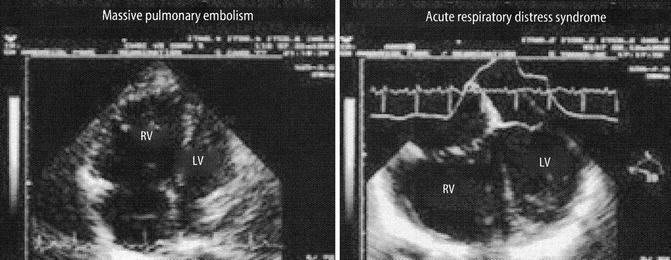
Fig. 53.5
Two examples of acute right heart dilatation affecting left heart compliance in acute pulmonary embolism and ARDS. The septum is shifted leftwards and encroaches on the LV Left ventricle cavity (From Jardin (2003))
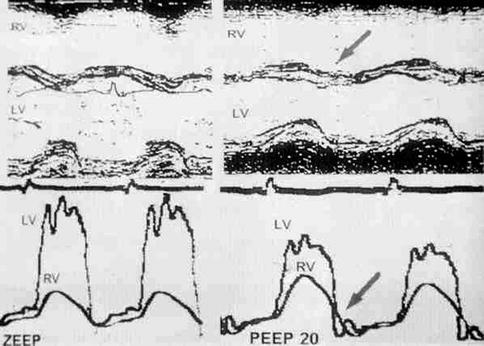
Fig. 53.6
Top: cardiac M-mode echo recording of septal wall motion before and after the application of 20 cmH2O PEEP. This produces paradoxical septal motion with leftward displacement at end systole (arrow). Bottom: simultaneous RV and LV pressure recordings which show reversal of the transseptal pressure gradient at end systole (arrow) and diastolic pressure equalization, maintaining the IVS in a shifted position (From Jardin et al. (1997))
Echocardiography has been a very useful tool in helping us understand more ventilation-induced changes in intrathoracic pressure and intravascular volume in patients with sepsis and ARDS. Investigators have used echo, pulsed Doppler, and interrogation of the arterial pulse pressure to evaluate changes in chamber dimensions, collapsibility of the great veins, and the response to volume loading (Jardin 1997, 1999, 2003, 2004; Jardin and Bourdarias 1997; Vieillard-Baron and Jardin 2003; Jardin et al. 1997; Vieillard-Baron et al. 1998, 1999, 2001a, b, c, 2002a, b, 2003a, b, c, 2004). It has long been recognized that arterial pressure rises during positive pressure lung inflation, a phenomenon sometimes referred to as reversed pulsus paradoxus (Jardin et al. 1983; Massumi et al. 1973). The changes in pulse pressure can be described as a succession of inspiratory increases followed by expiratory decreases (Jardin 2004). Studies in humans with sepsis and respiratory failure have shown that at peak inflation the LV stroke volume increases while that of the RV falls. The increase in alveolar pressure is associated with an increase in LA size and an augmented Doppler flow signal in the pulmonary veins as the capillaries are squeezed (Vieillard-Baron et al. 2003a). At the same instant RV stroke volume falls because of an increase in impedance (Vieillard-Baron et al. 1999), while the delay in refilling of the capillary bed results in a drop in arterial pulse pressure back to the pre-inspiratory level as the filling reserve of the LV falls in the next few cardiac cycles (Vieillard-Baron et al. 2003a; Preisman et al. 1997). In patients who are hypovolemic, there is a pronounced fall in arterial pulse pressure reflecting predominantly a reduction in RV preload which results in reduced LV stroke volume. The amplitude of the expiratory decrease has been used to detect fluid responsiveness (Michard et al. 2000; Tavernier et al. 1998). Vieillard-Baron and co-workers have also used echocardiography measurements of IVC and SVC size during inspiration and expiration to diagnose hypovolemia (Vieillard Baron et al. 2001; Barbier et al. 2004) and used a “collapsibility” index to help guide fluid administration in ventilated patients with sepsis (Vieillard-Baron et al. 2001b, c) (Fig. 53.7).
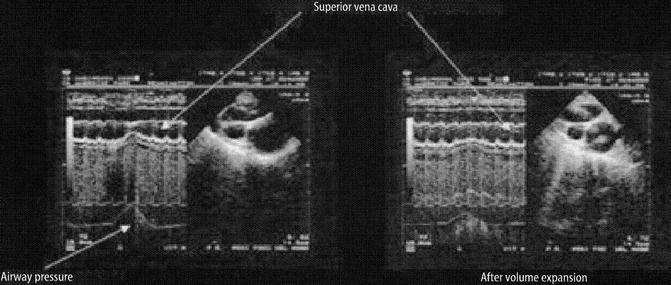
Fig. 53.7
Illustration of the effect of positive ITP on SVC flow in a patient before and after volume expansion. The SVC demonstrates “collapsibility” at peak inspiration prior to volume expansion (From Jardin (2003))
Similar principles that govern the increase ITP associated with PPV govern the use of noninvasive ventilation in ARDS. Depending on the underlying lung compliance, patients who are capable of maintaining adequate spontaneous ventilation in ARDS with use of CPAP may achieve better oxygenation for the same level of PEEP with less adverse hemodynamic effect and therefore better oxygen delivery due to the lower mean airway pressures (Schlobohm et al. 1981; Shah et al. 1977; Simonneau et al. 1982). Dhainaut et al. (1986) have observed that when patients were changed from spontaneous respiration to CPAP in ARDS, there is a decrease in right ventricular end-systolic and end-diastolic volumes, suggesting a fall in right ventricular afterload. Jardin et al. (1984) have observed the opposite effects in normal subjects without lung disease. The explanation for this discrepancy lies in when FRC is normal, pulmonary vascular resistance is at its lowest, and right ventricular afterload is minimal. The application of CPAP in normal individuals will increase lung volume above FRC and compress the extra-alveolar vessels thereby increasing right ventricular afterload. In ARDS FRC is considerably reduced and pulmonary vascular resistance is increased at these low lung volumes, a situation that can be reversed by the application of CPAP. Dhainaut has also been able to demonstrate during CPAP both cardiac output and oxygen consumption decrease but that with volume expansion cardiac output increases, while oxygen consumption remains low, suggesting decreased oxygen demand. They have suggested that the explanation for the reduced demand is the reduction of oxygen consumed by the work of breathing following the application of CPAP.
The benefit from application of PEEP to patients with severe ARDS could be viewed using a completing risks analysis, i.e., what is good for the lungs is not necessarily ideal for the heart and cardiovascular system. The use of high levels of PEEP as part of the “open lung” approach may recruit areas of atelectasis and improve both lung compliance and oxygenation, but none of the recently published large randomized trials have shown an improvement in survival (Meade et al. 2008; Mercat et al. 2008). Some insights into why this may be so can be gleaned from the classic study of PEEP in ARDS by Suter et al. published 35 years ago, which is worthy of detailed review (Suter et al. 1975). This was done at a time when there was a considerable amount of debate about the hemodynamic consequences of even a modest amount of PEEP. In this study 15 ventilated normovolemic adult patients with ARDS had PEEP applied in incremental levels of 3 cmH2O while lung compliance, intrapulmonary shunt, and dead space were measured. In addition, measurements of cardiac output, pulmonary venous saturation, and mixed venous oxygen saturation allowed oxygen transport to be calculated. PEEP was increased until there was a fall in cardiac output, which occurred at levels of between 6 and 18 cmH2O. They defined “best PEEP” to be the level which coincided with maximum oxygen transport and varied from patient to patient between 0 and 15 cmH2O (Fig. 53.8).
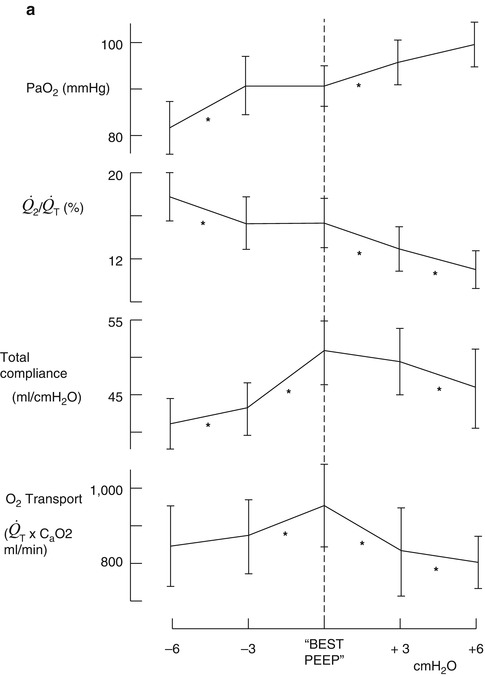
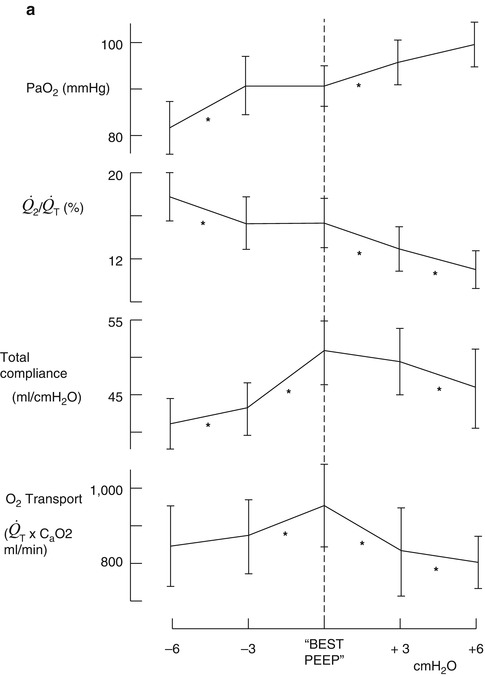
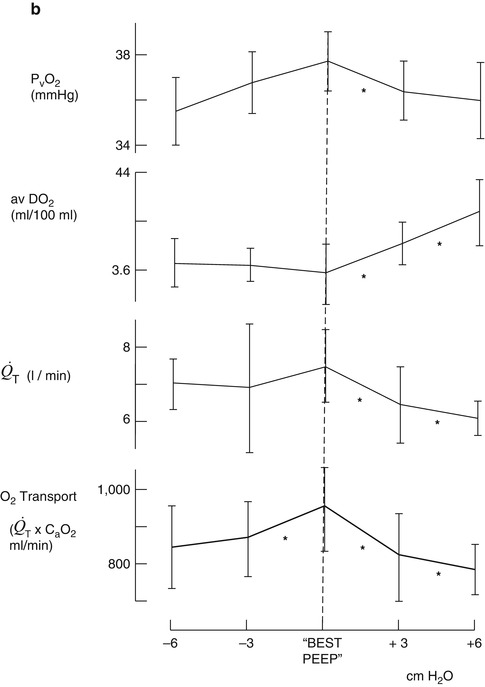
Fig. 53.8
(a, b) Change in PaO2, intrapulmonary shunt (
), total compliance, O2 transport (a) mixed venous oxygen tension (PvO 2 ), cardiac output (
), and arteriovenous oxygen difference (avDO 2 ) (b) at levels above and below “best PEEP” (From Suter et al. (1975))


The important message in this study needs to be relearned 35 years after it was first published, which is that ARDS is a multisystem disease with changes in lung function as its most obvious clinical manifestation. Treatment strategies, which are the ones adopted in clinical trials, have been lung focused emphasizing lung recruitment and minimizing lung distention by using low tidal volumes and high PEEP. Few, if any, have measured any index of the effect on cardiovascular function such as the mixed venous oxygen saturation (SvO2). At the end of the day, in a disease where multiorgan failure is an invariable component, it is all about oxygen delivery. This is the most important feature of all cardiopulmonary interactions.
53.4 The Effect of Changes in Intrathoracic Pressure in Congenital Heart Disease
53.4.1 Intrathoracic Pressure in Tetralogy of Fallot and Fontan Physiology
Both spontaneous and positive pressure ventilation have important cardiovascular effects in patients with heart abnormalities. One of the most important groups is children with obstructive right heart lesions such as tetralogy of Fallot and tricuspid and pulmonary atresia where changes in ITP can have a dramatic effect on pulmonary blood flow. Important new insights into that have improved our understanding of the cardiorespiratory physiology and how ventilation can change hemodynamics in these patients have come from a series of investigations done by Redington and colleagues. In patients with single ventricle and left atrial isomerism, studied remotely following total cavopulmonary anastomosis, they showed that there is significant augmentation of the pulmonary blood flow Doppler signal during the inspiratory (negative pleural pressure) phase of spontaneous respiration (Redington et al. 1991) (Fig. 53.9). The application of a Valsalva maneuver resulted in complete obliteration of the PBF, while the large negative pleural pressure produced by a Mueller maneuver gave rise to augmentation of the signal. A study on patients with a Fontan circuit found a 35 % augmentation of PBF during the inspiratory phase of spontaneous breathing (Penny et al. 1991; Penny and Redington 1991). They went on to make some important observations on the effect of changes in intrathoracic pressure and venous return and PBF in patients with tetralogy of Fallot. Diastolic right ventricular dysfunction is a common finding following surgical repair of severe right ventricular outflow tract obstruction and is characterized by a pulsed Doppler signal showing antegrade pulmonary artery flow during atrial systole accompanied by retrograde flow in the superior vena cava (Kisanuki et al. 1987; Redington et al. 1992). This is due to the fact that right ventricular end-diastolic pressure exceeds pulmonary artery diastolic pressure due to the stiffness of the right ventricle. There is premature opening of the pulmonary valve and the RV acts as a passive conduit between the right atrium and the pulmonary artery. In a study by Cullen et al. (1995) of postoperative tetralogy patients, half had this feature and those that did had a higher incidence of ascites and pleural effusions and longer durations of ICU stay. He also made the important observation that during the inspiratory phase of positive pressure ventilation, the Doppler signal of antegrade flow in the pulmonary artery was obliterated and there was a decrease in the flow signal across the tricuspid valve (Fig. 53.10). This gave rise to the speculation that negative pressure ventilation (NPV) might actually improve cardiac output and PBF in children following biventricular repairs. In an initial study, Shekerdemian and colleagues compared positive with negative pressure in otherwise healthy children undergoing catheterization and PDA closure with seven children in ICU who had undergone biventricular repair of CHD (Shekerdemian et al. 1997). They found that NPV was associated with a significant increase in cardiac output in the postoperative patients (Fig. 53.11). They did further series of acute studies in 11 patients after repair of tetralogy or the Fontan operation (Shekerdemian et al. 1996). Patients were switched from positive pressure to negative pressure ventilation for 15-min periods while cardiac output, PBF, and oxygen consumption were measured by the Fick equation and mass spectrometry. NPV was associated with a 46 % increase in PBF, a 48 % increase in stroke volume, and a 4.6 % increase in SVO2. Similar to the extended studies they had done in the Fontan patients, they did a series of short-term (15 min) and extended trials of NPV in 23 children who had undergone repair of tetralogy, 8 of whom had restrictive right ventricular physiology (Shekerdemian et al. 1999). These patients were characterized by antegrade diastolic pulmonary artery flow and had a more significant degree of metabolic acidosis compared with their nonrestrictive peers. By the end of 45 min of NPV, pulmonary blood flow had increased by 67 % in the group as a whole, but interestingly the trend to improvement was lower in the restrictive group. The beneficial effect was lost when patients were switched back to PPV.
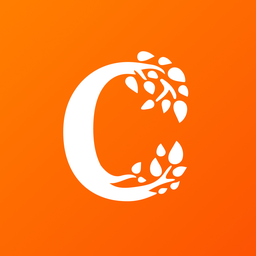
Full access? Get Clinical Tree
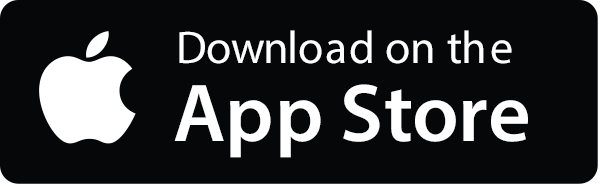
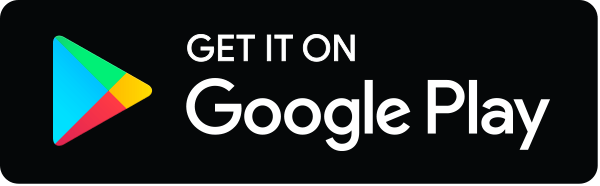