Pulse oximetry should be performed in every child who attends hospital with acute bronchiolitis
Infants with pulse oximetry oxygen saturation (SpO2) ≤ 92 % require inpatient care
Decision about infants with SpO2 between 92 and 94 % should be supported by detailed clinical assessment
Infants with SpO2 > 94 % in room air may be considered for discharge
Supportive therapies for those admitted to hospital
Nasal suction should be used to clear secretions in those with distress due to nasal blockage
Nasogastric feeding should be considered in infants who cannot maintain oral intake or hydration
Infants with SpO2 ≤ 92 % or who have severe respiratory distress or cyanosis should receive supplemental oxygen by nasal cannulae or face mask
Infants may be considered for discharge when they can maintain SpO2 > 94 % in room air and more than 75 % of their usual daily oral intake
Indication for acute assessment and high–dependency or intensive care referral
Failure to maintain SpO2 > 92 % with increasing oxygen therapy or cyanosis
Recurrent apnea
Poor feeding (<50 % in the preceding 24 h) or lethargy
Presence of nasal flaring and/or grunting
Severe chest wall recession
Increasing respiratory distress and/or exhaustion despite supplemental oxygen. Blood gas analysis may have a role in the assessment of infants with severe respiratory distress
50.3 Respiratory Failure in Bronchiolitis
A small proportion of cases of RSV bronchiolitis develop difficulties that are best managed in the PICU. Studies in the intensive care population suggest that 4–15 % of infants with RSV infection are previously healthy and 10–40 % are less than 36 weeks’ gestational age (Law et al. 1997; Carbonell-Estrany et al. 2004). In individual series, prematurity and bronchopulmonary dysplasia have significant impact, and such cases are likely to require either high-dependency or intensive care (Greenough et al. 2001). The prediction of who will require PICU admission is not an exact science. Brooks et al. (1999) found in a population of 542 previously healthy term infants, where 1.8 % required admission, that tachypnea (respiratory rate >80/min) and pulse oximetry arterial desaturation (SpO2 < 85 %) had good specificity (>97 %) but poor sensitivity (≤30 %) for predicting admission. The decision to admit to the PICU should depend on local referral practice and the ability to manage the problems outlined in Table 50.1.
50.3.1 Patterns of Disease Requiring Mechanical Ventilation
There are no absolute indications for controlled mechanical ventilation (MV). The clinical situation should dictate what is required. For example, if a patient is being transferred between hospitals, patient safety during transfer may be the overriding guiding factor. Alternatively, if a patient is being managed in a ward area within easy access of PICU practitioners, then it may be appropriate to wait until the infant demonstrates significant respiratory deterioration, such as those suggested by Rakshi and Couriel (1994):
-
Persistent apneas with severe hypoxemia and pH < 7.20
-
Altered mental state and SpO2 < 85 % with fractional inspired oxygen (FiO2) above 0.6
The mechanism leading to progressive respiratory failure and apparent exhaustion will be discussed in later sections of the chapter (see Sects. 50.3.2 and 50.3.3). The other patterns of disease requiring MV are peripheral circulatory collapse, recurrent apneic attacks, and generalized convulsions (Simpson et al. 1974; Eisenhut 2006). Recurrent apnea in an infant, in the winter, should lead to the early diagnosis of bronchiolitis at the time of presentation. However, the diagnosis of underlying RSV infection may be overlooked in cases presenting with shock or hyponatremia-related seizures.
Apnea is common in RSV infection, particularly in those with a history of apnea of prematurity; it is usually nonobstructive (Anas et al. 1982). The mechanism of RSV-associated apnea is unclear, but possible explanations include stimulation of laryngeal chemoreceptors, sensitization of airway receptors, respiratory muscle fatigue, and abnormal immunologic responsiveness.
The shock-like state complicating RSV has been described by a number of authors (Simpson et al. 1974; Njoku and Kliegman 1993; Kim and Frankel 1997; Arnold et al. 2000; Checchia et al. 2000). There are some infants where the respiratory features of RSV infection are silent at presentation but can become severe enough to require high-frequency oscillatory ventilation (HFOV) (Arnold et al. 2000). There are others, where cardiac compromise is a consequence of cardiopulmonary interaction that improves with clinical improvement. For example, Sreeram et al. (1991) studied 21 infants with acute bronchiolitis, with normal hearts, and found that half had tricuspid valve regurgitation; many had evidence of raised pulmonary artery systolic pressure. In all such patients, myocardial injury may contribute to the severity of presentation (Checchia et al. 2000).
Seizures during RSV infection usually result from hyponatremia (Simpson et al. 1974; Hanna et al. 2003), which is a consequence of the derangement in water, electrolyte, and endocrine homeostasis induced by high intrathoracic pressure (Gozal et al. 1990; Poddar et al. 1995). Typically, seizures cease once serum sodium is above 130 mmol/L.
50.3.2 Blood Gases and Respiratory Failure
Respiratory failure is not always present on admission to hospital, and progressive deterioration over a variable time period occurs in many such cases, which confirms the importance of clinical vigilance even in mild to moderately severe cases. Both components of respiration – carbon dioxide (CO2) clearance and oxygenation – need to be assessed in infants with RSV infection.
In regard to CO2 derangements in bronchiolitis, respiratory failure is defined as an arterial partial pressure of CO2 (P aCO2) exceeding 65 mmHg (8.7 kPa). Reynolds (1963a) found that when such infants breathed air, there was little increase in P aCO2 above 40 mmHg, even when respiratory rates were up to 60 breaths/min (Fig. 50.1). At a breathing rate of 60 breaths/min, the arterial partial pressure of oxygen (P aO2) was ~60 mmHg (8 kPa). Downes et al. (1968) found two phases or a progression in CO2 clearance. In infants with P aCO2 below 65 mmHg, the dead space-to-tidal volume ratio (V D/V T) was between 0.55 and 0.69. (In normal, spontaneously breathing infants, the ratio is 0.25 ± 0.07, which is similar to that found in adults.) When increase in V D/V T originates within the respiratory system, it represents increase in V D, decrease in V T, or some combination of the two. Krieger and Whitten (1964) found lower mean V T and mean minute volume in 24 infants with acute bronchiolitis; they concluded, “respirations may be considered shallow.” Distinct from these causes, outside of the respiratory system, hypovolemia or shock may also lead to high V D/V T. Given this limit to lung physiology in bronchiolitis, avoidance of respiratory failure can be accomplished by maintenance of two- to threefold increase in minute volume (Downes et al. 1968). In bronchiolitis, if V T is limited, then compensatory increase in minute ventilation has to be due to an increase in respiratory frequency (Stokes et al. 1981). It is interesting to note that infants with respiratory failure (based on clinical and blood gas findings) have high V D/V T associated with minute ventilation close to predicted basal levels, which implies that the needed increase in minute ventilation has not been maintained. At this point, some infants are extremely tachypneic, so their deterioration represents worsening increase in V D or lessening of V T. In other infants, this state represents fatigue or an abnormality in lung mechanics that cannot be overcome. Of note, the V D/V T remains raised (0.46 ± 0.10) even after starting MV, which is due to increase in physiologic dead space caused by hyperinflation or associated hypoperfusion (Almeida-Junior et al. 2007).
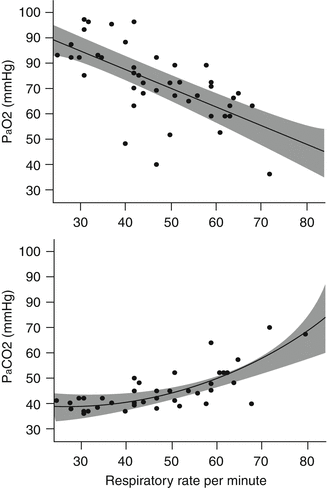
Fig. 50.1
Partial pressure of oxygen (P aO2) and carbon dioxide (P aCO2) plotted against respiratory rate in infants with bronchiolitis who are spontaneously breathing room air (Figures redrawn from Reynolds (1963a) with additional regression analysis and 95 % confidence interval for fitted lines)
Clinical assessment of oxygenation – particularly hypoxia – is difficult in bronchiolitis (Simpson and Flenley 1967). Cyanosis is invariably present when SpO2 is below 85 %, but this is a late sign. There is a significant inverse relationship between respiratory rate and P aO2 (Reynolds 1963a). In those breathing air, respiratory rate increases from 25 to 60 breaths/min as P aO2 falls from 90 to 60 mmHg (Fig. 50.1). There is also a significant inverse linear correlation between P aCO2 and P aO2, which suggests that alveolar hypoventilation contributes to arterial hypoxemia (Downes et al. 1968). From first principles, in the Downes et al. data, the degree of hypoxemia is as expected from the alveolar gas equation and a normal alveolar-arterial oxygen (AaDO2) gradient: at a time when P aCO2 is 65 mmHg (and assuming a respiratory quotient of 0.8), the expected alveolar PO2 is 68 mmHg, which is consistent with the P aO2 of 50 mmHg observed. However, Reynolds (1963b) found much higher AaDO2 in 10 infants with bronchiolitis, ranging from 35 to 57 mmHg, and concluded that since administering oxygen (fractional inspired oxygen, FiO2, 0.4) made their P aO2 rise to above 100 mmHg (13.3 kPa), the most likely cause of large AaDO2 gradient was ventilation-perfusion inequalities. As the condition becomes extremely severe, intrapulmonary shunt may become the more significant cause of high AaDO2 (Tasker et al. 2000).
Taken together, it is likely that abnormal gas exchange in bronchiolitis is produced by rise in V D/V T and worsening ventilation-perfusion inequalities. In less severe states, the former is compensated by increase in minute ventilation. In more severe disease, there is failure in this compensation. The critical questions are as follows: what is driving tachypnea, and what is causing V D/V T to increase? Rising P aCO2 is not the stimulus for rapid breathing, since respiratory rate is increased despite near-normal levels. Neither is P aO2 the stimulus, since this parameter increases respiratory rate when <60 mmHg.
50.3.3 Lung Function and Mechanics in Bronchiolitis
The total work that has to be performed on the lung proper is the work necessary to overcome both elastic and nonelastic resistance. In addition to elastic and nonelastic resistance, respiratory work is a function of rate and V T. Work of breathing in bronchiolitis is double to six times that of normal infants but similar to that of infants with bronchopneumonia (Krieger and Whitten 1964; Stokes et al. 1981). Interestingly, the latter do not present in extremis, which implies that this increase in work of breathing can be tolerated, but the problem may lie in whether lung mechanics in bronchiolitis permit such work. Since individual clinical features (e.g., auscultation, respiratory rate, and heart rate) do not correlate with total work of breathing, it is difficult to assess (Stokes et al. 1981). The degree of hyperinflation may be helpful in the assessment because it does mirror severity, but the best measure of work is esophageal pressure changes during breathing.
Given the obstructive lesions in terminal bronchioles in bronchiolitis, we would expect high nonelastic resistance. Yet, in general, infants with bronchiolitis do not show prolongation of the expiratory phase. They also breathe rapidly, which is inefficient when there is increased resistance to airflow. In one series of acute bronchiolitis, mean nonelastic resistance was similar to that of normal infants of similar age distribution (~0.03 cm H2O/mL/s) (Krieger 1964). This finding can be explained by the theory of “equality of time constants,” i.e., when flow is rapid in a system of unequally obstructed airways, the measured resistance will approximate that of the large peripheral pathways (Otis et al. 1950).
In contrast to the normal findings in nonelastic resistance, elastic resistance is high in bronchiolitis, which also means that compliance is markedly decreased (Krieger and Whitten 1964; Panitch et al. 1993; Smith et al. 1993). This decrease in compliance is due, in part, to rapid respiratory rates (Helliesen 1958) and bronchiolar inflammation. The most significant factor, however, is the increased retractive force that is active at a high level of lung inflation; the degree of lung distention (i.e., V T) influences work expenditure more significantly when compliance is low. (Speed of distention, i.e., respiratory rate, is a more important influence when airflow resistance is increased.) Krieger and Whitten (1964) found that elastic work was less than expected in bronchiolitis. The rapid shallow mode of breathing, therefore, appears to be economical in terms of work expenditure when compliance is low (Otis et al. 1950), because elastic work is conserved through breathing fast. These points are illustrated in the two cases of bronchiolitis depicted in Fig. 50.2. The relationship between elastic (1/compliance), viscous (resistance), and total work of breathing per minute to frequency of breathing during bronchiolitis and on recovery is shown in graphs that have been calculated from first principles according to the equations described by Otis et al. (1950). The acute and convalescent data are from two cases of bronchiolitis reported by Krieger and Whitten (1964). The upper two panels (A and B) are from a 5.5 kg infant with, predominantly, reduced compliance. The lower two panels (C and D) are from a 4.5 kg infant with a mixed abnormality in compliance and resistance. The acute graphs (A and C) show a doubling of total work, due mainly to increased elastic work. The vertical lines show the infant’s respiratory rate and where this rate intersects the work curves.
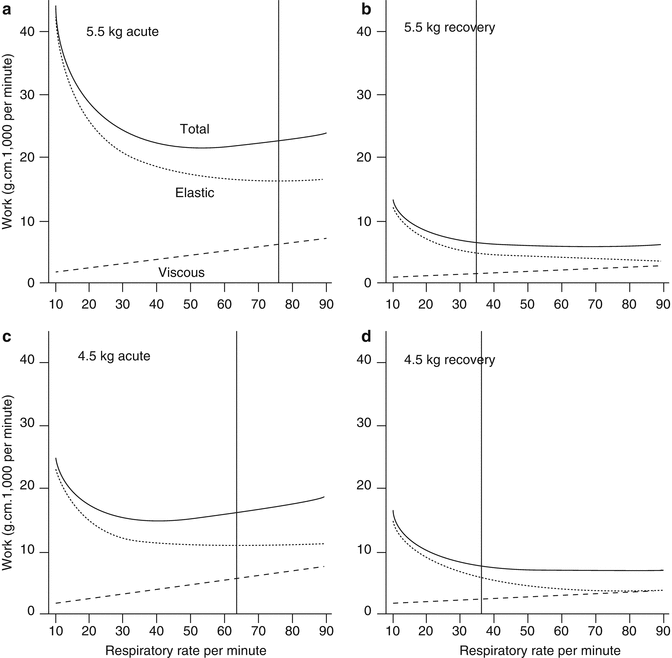
Fig. 50.2
Relationship of elastic, viscous, and total work of breathing per minute to frequency of breathing, during the acute and recovery phase of bronchiolitis. Graphs a and b refer to a 5.5 kg infant; graphs c and d refer to a 4.5 kg infant. The data are calculated according to the equations derived by Otis et al. (1950) using real data from two infants reported by Krieger and Whitten (1964) and assuming V D/V T changes from acute to recovery, of 0.45–0.25 (see Almeida-Junior et al. 2007). In the acute graphs (a and c), total work of breathing is increased compared with the recovery phase. Elastic work decreases with increasing respiratory rate, and viscous work increases with increasing respiratory rate. It is more efficient to breathe at higher respiratory rate during bronchiolitis as demonstrated by the vertical lines that show the breathing rate of the infant during each stage of illness. The recovery graphs (b and c) show optimum respiratory rate as lung mechanics improve
“Exhaustion” in infants with bronchiolitis does not result from extreme work during breathing. It is more likely a phenomenon of inability to perform the workload necessary for breathing using hyperinflated lungs. In bronchiolitis, hyperinflation places the muscles of inspiration at a mechanical disadvantage; they are unable to shorten sufficiently to produce the necessary reduction in intrapleural pressure. Another physiologic problem that is considered to follow from obstructive breathing with large negative intrathoracic pressure swings (unbalanced by similar positive pressure efforts) is transpulmonary fluid shifts and the development of pulmonary edema (Stalcup and Mellins 1977). Stokes et al. (1981) did not find evidence for this phenomenon in 26 hyperinflated infants with bronchiolitis. Instead, the esophageal pressure monitoring in these infants showed positive values for a large portion of the respiratory cycle, which suggests that positive pressures were produced on expiration. Fluid flux across the alveolar-capillary membrane would be avoided in this state because of limited hydrostatic pressure gradient.
50.4 Respiratory Support for Bronchiolitis
The lung abnormalities in RSV bronchiolitis are hyperinflation, impaired minute ventilation, impaired ventilation-perfusion matching, and an increase in physiologic dead space. As a consequence, the infant breathes rapidly and has increased elastic and viscous work of breathing. By the time that respiratory support is considered, hypercarbia and hypoxemia are also present. In this setting, the aim of respiratory support is to overcome the exaggerated work of breathing and abnormal lung mechanics and allow for resolution of the primary pathophysiology.
Most infants with respiratory failure have “obstructive” lung mechanics, but abnormality in compliance often coexists (Fig. 50.2). The discussion in previous sections (see Sects. 50.3.2 and 50.3.3) indicates that infants with progressive deterioration exhibit a continuum with lessening reserve and compromised homeostasis. A reasonable question, then, is to ask whether this progression can be limited. For example, it is the interaction between the patient’s drive to breathing and abnormal lung mechanics that leads to deterioration and ultimately respiratory failure. So why not intervene early to limit the effect of interaction between drive to breathing and lung mechanics? Altering lung mechanics or altering the effect of interaction between spontaneous breathing and lung mechanics could achieve this result.
50.4.1 Noninvasive Respiratory Support
Sixteen percent of infants hospitalized for RSV have apnea, and its course is usually short-lived. Clinically, these episodes are diaphragmatic or nonobstructive with complete absence of respiratory effort. In these cases, most clinicians would consider that endotracheal intubation with minimal ventilatory support is required until the problem resolves. However, McNamara and Sullivan (1997) reported an improvement in RSV-infection-related apnea with the use of nasopharyngeal prong continuous positive airway pressure (CPAP) alone.
We now know that patients with worsening respiratory distress due to hyperinflation can be supported without using endotracheal intubation. To date, three ways of delivering CPAP have been applied and reported:
-
Heated, humified, high-flow nasal cannulae (HFNC)
-
Nasopharyngeal CPAP
-
Nasopharyngeal bilevel positive airway pressure (BIPAP)
CPAP maintains positive transpulmonary pressure during spontaneous breathing. It keeps the airways open by moving the equal pressure point more proximal in the airways, such that airway collapse does not occur. It increases secretion clearance. It improves compliance and reduces the work of breathing. Last, it improves gas exchange. However, the cost of these benefits is that its use may lead to lung overinflation.
50.4.2 Heated Humidified High-Flow Nasal Cannulae
HFNC is a relatively new therapy that allows the delivery of high inspired gas flows (1–8 L/min in infants) with or without increased oxygen concentration (McKiernan et al. 2010). Ideally, these devices should deliver flow greater than the patient’s peak inspiratory demand to fully support their minute ventilation. In addition, HFNC provides some level of CPAP, which depends on the flow delivered relative to the size of the patient and on the leak around the nasal cannulae. Flows of 3–5 L/min in term infants have been shown to generate intrapharyngeal pressure of up to 5 cm H2O (Spence et al. 2007). The advantages of this system over conventional nasal CPAP delivery devices are that it is less cumbersome, better tolerated, and as a consequence used earlier. The disadvantage of this system is that not all attendants may realize that HFNC is CPAP by another name – it isn’t just nasal oxygen. As a consequence, clinical deterioration while receiving HFNC means failed low-level CPAP. McKiernan et al. (2010) reported their PICU experience of managing infants with RSV bronchiolitis before and after the introduction of HFNC to their practice. In the season after the introduction of HFNC, only 9 % of infants required intubation, compared with 23 % in the previous season (P = 0.04). HFNC decreased respiratory rate to a greater extent than other devices, and those with the greatest decrease in respiratory rate were least likely to be intubated. The authors also found that median PICU length of stay for cases of bronchiolitis decreased from 6 to 4 days.
50.4.3 Nasal CPAP
We know that in very preterm neonates, early nasal CPAP at birth reduces the number of days of MV (Morley et al. 2008). We also know that application of CPAP to nasal mask better reduces work of breathing when compared with the effect of CPAP applied to an endotracheal tube (Keidan et al. 2000; Pandit et al. 2001). This difference is most likely due to improved patency of the upper airway, rather than major increases in functional residual capacity (FRC) and lung compliance. In view of this theory, we should expect that early intervention with CPAP in bronchiolitis should reduce the work of breathing and may even obviate the need for endotracheal intubation and more invasive support.
Beasley and Jones (1981) reported the use of nasal CPAP early in the course of acute bronchiolitis in 14 infants. They found it improved gas exchange and was free of complications. Thia et al. (2008) showed, in a randomized controlled trial, that there is indeed a reduction in P aCO2 when using CPAP. This reduction was better if CPAP was used first than if it was used after a 13-h period with standard treatment. The success rate of noninvasive support in bronchiolitis found in other studies varies from 75 to 83 % (Soong et al. 1993; Campion et al. 2006; Larrar et al. 2006).
50.4.4 BIPAP
Javouhey et al. (2008) reported an approach that bridges the step-up in support between noninvasive airway pressure support and assisted ventilation. These investigators used noninvasive ventilation as their primary form of respiratory support in 27 infants with bronchiolitis. Their initial care was CPAP at 5–10 cm H2O. If this support failed, they progressed to BIPAP starting at 12 cm H2O and, thereafter, gradually increasing to 18 cm H2O with a backup rate at 30 breaths/min. Compared with their previous experience of similar severity infants, this PICU found that the rate of endotracheal intubation was significantly lower (89 % vs 52 %, P < 0.01) and, not surprisingly, the rate of ventilator-associated pneumonia was also much reduced.
50.5 Invasive Conventional Mechanical Ventilation
Mechanical ventilation in RSV bronchiolitis has as its goal patient stability; restoring adequate gas exchange and unloading the work of respiratory muscles bring about this state. The problem is that we are constrained by abnormal lung mechanics and the natural history of the condition. The current practice of respiratory support for bronchiolitis is largely based on clinical judgement and local experience, rather than any consensus (Leclerc et al. 2001). Yet despite this source for variation, the time course of admission on the PICU is remarkably consistent. Cases with no preceding or underlying disease intubated for apnea or respiratory deterioration will require MV for 4–5 days, on average. Cases with preceding disease are ventilated much longer, on average for up to 3 weeks (Stretton et al. 1992; Tasker et al. 2000).
50.5.1 Initial Settings for Mechanical Ventilation
A review of MV settings applied to infants with RSV – reported in the literature 1967–1994 – reveals considerable variation in practice (Table 50.2). Both volume-cycled and pressure-cycled MV are described in the literature with breathing rates varying from 10 to 60 breaths/min, maximum pressures from 20 to 50 cm H2O, and V T from 6 to 20 mL/kg (Leclerc et al. 2001). There is no evidence for a superior mode of MV, and it would be difficult to plan such a study since mortality and morbidity are low and, as noted above, time course on PICU is fairly consistent. We are therefore left with a pragmatic approach that takes account of the prevailing pathophysiology. Figures 50.3 and 50.4 illustrate this point. The inflation-deflation volume curves above FRC are hypothetical for a model 5 kg infant and based on data presented in the literature. (The curves are a simplification of the ideal situation with a number of assumptions – see figure legend – but they do illustrate the physiology). The “control” cases in Fig. 50.3 represent the mean data for controls in the report by Hammer et al. (1997): if one chose to ventilate a 5 kg infant at a rate of 30 breaths/min with inflation pressure (P inf) of 16 cm H2O, we may expect P aCO2 35 mmHg. The “bronchiolitis” cases in Fig. 50.3 represent the mean data for the unselected cases of bronchiolitis reported by Derish et al. (1998). If we make the assumption that there is an increase in V D/V T ratio during bronchiolitis (Almeida-Junior et al. 2007) as well as a change in respiratory system compliance (C rs) and resistance (R rs), continuing to ventilate a 5 kg infant at a rate of 30 breaths/min with P inf 16 cm H2O will result in P aCO2 46 mmHg (graph A). Note that the inspiratory time (t i) has been increased to 0.50 s to allow inflation lung volume above FRC to reach 40 mL (i.e., 8 mL/kg). If the t i had remained the same as in “controls,” inflation lung volume above FRC would only be 3 mL/kg. Graph B shows the potential danger of responding to P aCO2 46 mmHg with simultaneous increases in P inf to 20 cm H2O and ventilator frequency to 36 breaths/min. Lung volume above FRC rises to 10 mL/kg, and P aCO2 falls to hypocapnic level (i.e., 31 mmHg).
Table 50.2
Ventilator settings used in infants with RSV (1967–1994)
Variable |
Value |
---|---|
Ventilator rate (breaths/minute) |
10–15 to 30–60 |
Maximum peak inspiratory pressure (cm H2O) |
20–25 to 30–50 |
Positive end-expiratory pressure (cm H2O) |
3–15 |
Tidal volume (mL/kg) |
6–20 |
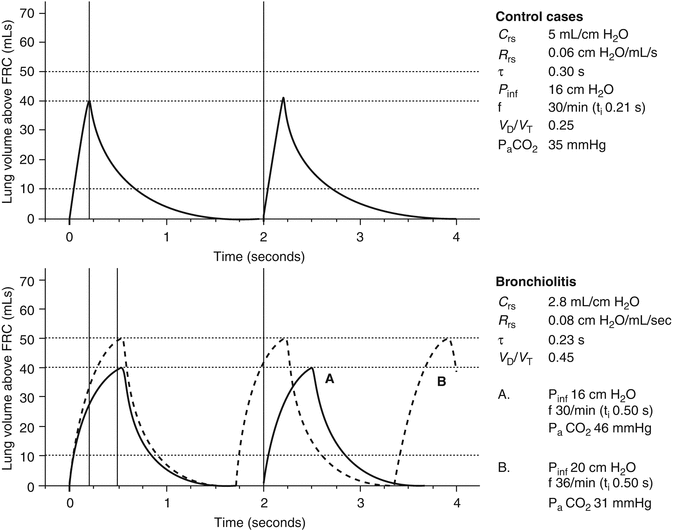
Fig. 50.3
Calculated time course of inflation and deflation curves for artificial ventilation by intermittent application of constant pressure (square wave) followed by passive expiration. The graphs are normalized for a model 5 kg infant and assume full sedation and neuromuscular blockade, where C rs is respiratory system compliance, R rs is respiratory system resistance, τ is the time constant, P inf is the inflating pressure, f is the ventilation frequency, t i is the inspiratory time, and V D/V T is the dead space-to-tidal volume ratio. The “control” cases are the 26 controls in the series reported by Hammer et al. (1997). The “bronchiolitis” cases are the 25 cases reported by Derish et al. (1998). P aCO2 is estimated from the available data and assumes weight of 5 kg and constant respiratory quotient (0.86) and CO2 production across groups. See text for details. The definitions of curves A and B are shown in the column
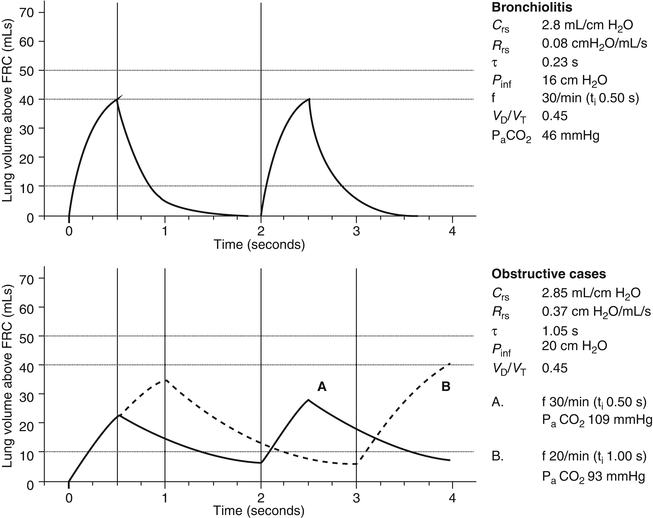
Fig. 50.4
Calculated inflation and deflation curves for “bronchiolitis” and “obstructive” cases. The nomenclature and assumptions are the same as Fig. 50.3. The “bronchiolitis” cases are the 25 cases reported by Derish et al. (1998). The “obstructive” cases are the 27 selected cases reported by Hammer et al. (1997). See text for details. The definitions of curves A and B are shown in the column
Figure 50.4 shows the “bronchiolitis” data from Derish et al. (1998). The upper panel is the same as graph A in Fig. 50.3 and is used for comparison. The “obstructive” cases represent the mean data for the cases selected as “obstructive” bronchiolitis reported by Hammer et al. (1997). These cases had more severe disease than those reported by Derish et al. (1998). The main difference is that the cases reported by Derish et al. (1998) have an isolated fall in C rs, whereas the cases reported by Hammer et al. (1997) have a fall in C rs and increase in R rs. Graph A shows the inadequacy of ventilation at a rate of 30 breaths/min and a t i (0.50 s) similar to that for the “bronchiolitis” cases, even though the P inf is increased to 20 cm H2O. The estimate of P aCO2 is now 109 mmHg. The deflation curve also shows the development of dynamic hyperinflation (see end-expiratory volume above zero). Graph B shows the effect of lengthening t i and decreasing the ventilator rate. The problem of dynamic hyperinflation continues, but tidal volume is improved. P aCO2 is nowhere near being fully controlled (i.e., 93 mmHg), but further increase of P inf, lengthened t i, and slower ventilator frequency will bring P aCO2 to more tolerable levels.
These examples highlight the clinical reality of ventilatory management of bronchiolitis. In less severe cases, with modest decrease in C rs, standard approaches should not cause harm providing ventilator rates are limited to 30 breaths/min. In more severe cases, in the absence of lung function testing, clinical observation of inspiratory and expiratory chest excursion as well as regular auscultation is important. The aim in the “obstructive” lung is to avoid overventilation, with associated hyperinflation, and barotrauma. Ventilatory management should therefore take account of two guiding principles:
-
Achieve adequate but not necessarily normal arterial blood gases. Adequate oxygenation is considered present with P aO2 > 60 mmHg (8 kPa) or SpO2 > 88 %. Adequate minute ventilation is considered present with any value of P aCO2 that achieves pH > 7.25 (so-called permissive hypercapnia strategy; see Sect. 50.6.1).
-
Avoidance of overdistention of hyperinflated lungs by limiting V T to 6–8 mL/kg. This goal may even necessitate MV at slow ventilation rates (10–15 breaths/min) with prolonged expiratory times to limit ventilator-associated dynamic hyperinflation and impaired minute ventilation. (MV of “restrictive” lungs is discussed in Sect. 50.6). Our recommendation is that ventilator rate should be limited <30 breaths/min.
All infants will require adequate analgesia and sedation during MV. Morphine (20–40 μg/kg/h), chloral hydrate (30–50 mg/kg every 4 h), and midazolam (50–250 μg/kg/h) are commonly used. In combination, these drugs will alter the CO2-ventilatory response, with a decrease in the slope and an increase in the apnea threshold (Yaster et al. 1990). These features are useful when applying the permissive hypercapnia strategy (see Sect. 50.6.1). However, these effects may be detrimental after extubation since the expected protective response to worsening gas exchange will be blunted, hence the need for continued close observation 12–24 h after extubation (see 4.5.3). When neuromuscular blockade is required, vecuronium (50–100 μg/kg/h) can be used. The main reason for neuromuscular blockade is to enable MV at either very slow rates or fast (HFOV) rates and prevent ventilator-patient asynchrony. As will be discussed later, neuromuscular blockade is not necessarily required during HFOV (see Sect. 50.6.2).
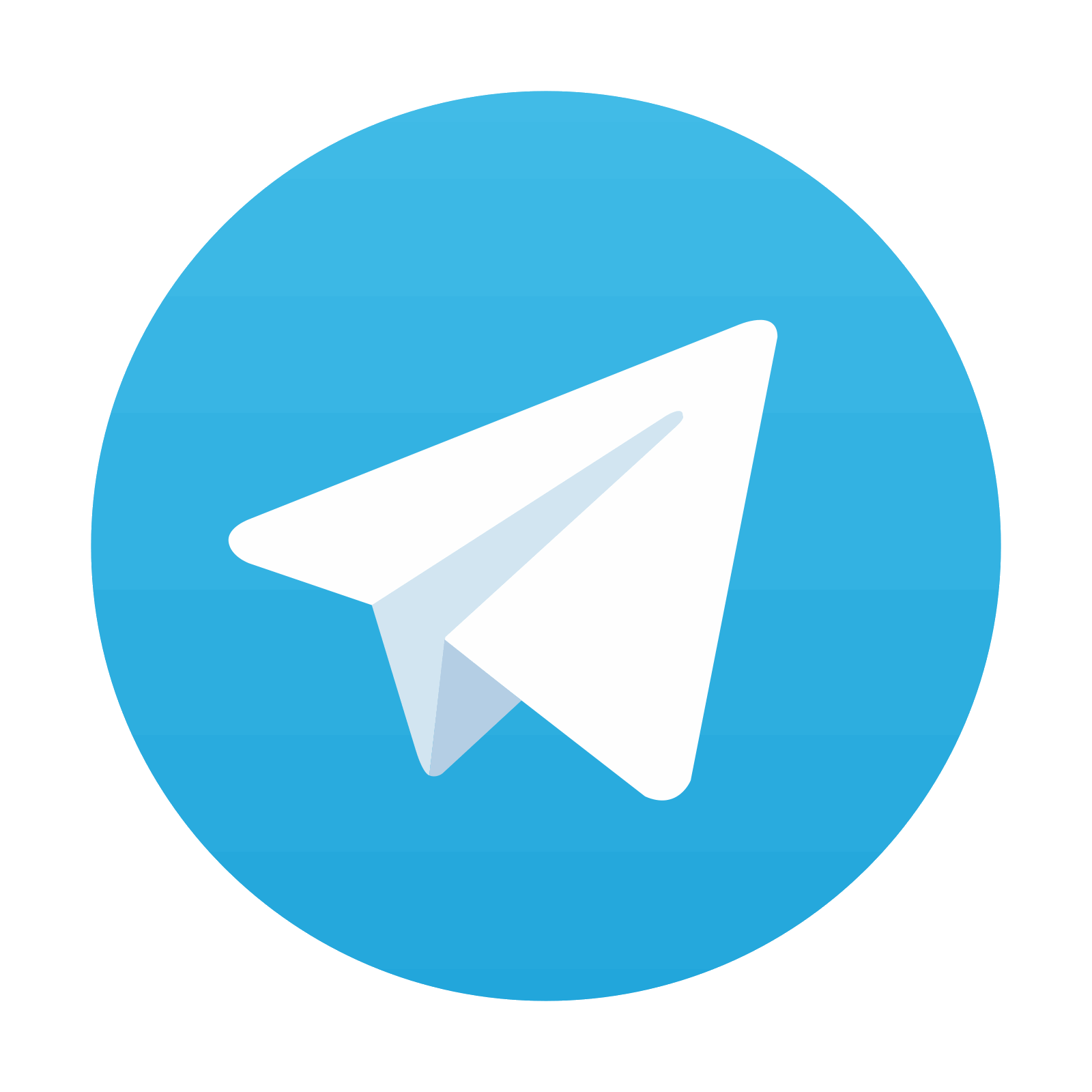
Stay updated, free articles. Join our Telegram channel
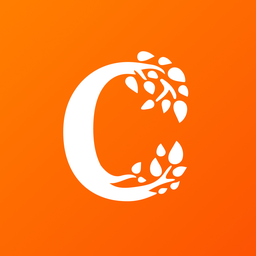
Full access? Get Clinical Tree
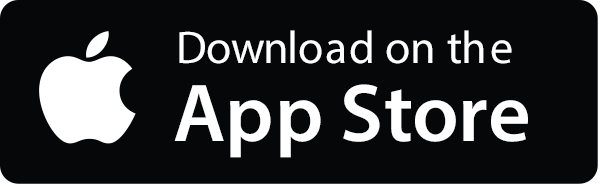
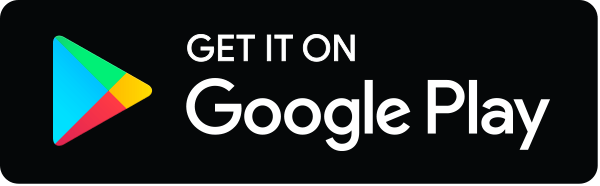