Biotransformation of Medications by Human Placenta
Olga Zharikova
Tatiana Nanovskaya
Mahmoud S. Ahmed
Introduction
Human placenta is a tissue of fetal origin located at the interface between the maternal and fetal circulations. The structure and diverse functions of human placenta undergo changes with gestation to accommodate and ensure normal fetal growth and development. Through gestation, the placenta assumes the functions of several organs that include the exchange of gasses (lungs), uptake of nutrients (gut), elimination of waste products (kidneys), and others. Other functions, such as the biosynthesis of human chorionic gonadotropin (hCG) and human placental lactogen (hPL), are specific to trophoblast tissue.
Historically, the placenta has been viewed as a protective barrier that decreases fetal exposure to endogenous metabolic waste products, xenobiotics, environmental pollutants and toxins (1). However, this view, following instances of birth defects related to the administration of medications/drugs to pregnant patients, was revised and the placenta was considered as a passive organ which allows molecules to indiscriminately pass across to the fetal circulation (2). For that reason, one of the major concerns of health care providers and researchers is the extent to which the placenta could protect the fetus from exposure to the medications that are administered to a patient during pregnancy. Current information indicates that human placenta “regulates” fetal exposure to xenobiotics by the existence of trophoblast/basal membrane layers and the activity of its metabolic enzymes responsible for the biotransformation of endogenous and exogenous compounds as well as the activity of its efflux transporters that extrude these compounds from the tissue and fetal circulation back to the maternal circulation.
This chapter will focus on the placental enzymes that are responsible for the biotransformation of medications administered to the pregnant woman. Several of these enzymes also catalyze reactions in metabolic pathways for the biosynthesis of important compounds. In this chapter, the term “biotransformation” will be used to describe the conversion of a medication/drug to its metabolite(s) and the term “metabolism” for conversion of one endogenous compound to another.
Overview of Xenobiotics Biotransformation
Human liver is the primary organ responsible for the metabolism of endogenous compounds and the biotransformation of xenobiotics including drugs/medications. Other organs which are involved in the biotransfomation of medications are the intestine, pancreas, lungs, brain, skin, mammary glands, kidneys, testes, prostate, uterus, ovary, and the placenta (3,4,5,6,7,8,9,10,11).
The hepatic and extrahepatic enzymes responsible for the biotransformation of medications are classified according to the type of reaction they catalyze as well as their involvement in either phase I or II metabolism. The largest class of enzymes responsible for the biotransformation of medications and other compounds is the cytochrome P450 (CYP450) family of isozymes. The biotransformation of medications by CYP isozymes results in the formation of a metabolite that is either pharmacologically active, inactive (12), or toxic (13,14). The metabolites formed during phase I can be eliminated from the body unchanged or could be conjugated with the endogenously derived glucuronic acid, sulfate, glutathione, amino acids, or acetate (phase II) to form water-soluble conjugates (15). Most of these conjugated compounds are pharmacologically inactive and are rapidly excreted.
Determining the Activity of Enzymes Responsible for the Biotransformation of Medications
Information on the activity of hepatic and extrahepatic tissue enzymes responsible for the biotransformation of a medication is essential for the better understanding of its pharmacokinetics (PK) and pharmacodynamics (PD).
The placental enzymes are mainly localized in the endoplasmic reticulum of trophoblast tissue (microsomes), in the mitochondrial membranes, and to a lesser extent in the brush border membranes and cytoplasm. The expression of these enzymes in the tissue can be determined in vitro at the protein, mRNA (messenger RNA), or DNA levels. However, the presence of an enzyme as determined by its expression does not reflect its activity.
The activity of an enzyme can be determined in vivo and or in vitro. In vivo, the activity is determined by the administration of a probe compound that is a specific substrate of the enzyme. A change in enzyme activity will result in altering the PK of the probe substrate and will be revealed by the change in the area under the time-concentration curve (AUC) (25). However, this approach cannot differentiate between the activities of hepatic versus extrahepatic (including placental) enzymes.
The metabolic activity of a placental enzyme can be determined only by in vitro reactions utilizing subcelleular fractions (mitochondrial, microsomal or cytosolic) prepared from postpartum trophoblast tissue homogenates. The data obtained provides information on the kinetic parameters of the reaction, that is, its maximum rate/velocity (Vmax) and the affinity of the substrate/medication to the enzyme (apparent Km). Another valuable parameter, for characterization of the enzyme activity, is its intrinsic clearance (Clin), which is calculated from Km/Vmax. The intrinsic clearance of an enzyme can be used for comparing its activities between tissues or the activity of two different enzymes in the biotransformation of the same substrate.
In addition, in vitro experiments allow the identification of the enzymes involved in the biotransformation of xenobiotics. The identification of enzyme(s) in a subcellular fraction (e.g., microsomal or mitochondrial) can be achieved by utilizing inhibitors of its activity. These inhibitors are chemicals that are selective/specific for the enzyme catalyzing the reaction and antibodies raised against these enzymes. The chemical or antibody causing the greatest inhibition suggests the identity of the enzyme involved. Confirmation of enzyme identification is achieved by comparing of the values of the kinetics parameters of the reaction catalyzed by the microsomes with those catalyzed by the cDNA (complementary DNA) expressed/recombinant or the purified enzyme. The above is true for the identification of the enzyme in question whether in placental or any other tissue preparation.
Regulation of Enzyme Activity
The activity of metabolic enzymes is regulated at the DNA, mRNA, and/or protein level and varies between different cells and tissues. In human placenta, the expression and activities of its enzymes varies with gestational age to accommodate the diverse requirements of the fetus during organogenesis and development (26,27,28,29,30,31). Consequently, it was assumed that several CYP isozymes expressed during embryogenesis could be “switched off” during the period of fetal development (32,33).
The placenta contains both constitutive and inducible CYP isozymes. The constitutive enzymes are expressed and their activity could be detected during most of the gestational period. On the other hand, the expression and activity of inducible enzymes are subject to an individual’s exposure to environmental and/or endogenous stimuli. An induced enzyme exhibits a transient increase in its activity for variable periods of time but usually returns to its basal level when the stimulus ceases to exist. The increase or decrease in the activity of an enzyme is referred to as enzyme up- or down-regulation and occurs at two levels namely, transcriptional (DNA to mRNA) or translational (mRNA to protein).
Enzyme Activation
The in vivo induction of a hepatic enzyme increases its activity and results in enhancing the biotransformation of a medication and consequently decreasing its efficacy (12,34,35). A similar in vivo induction of a placental enzyme that catalyzes the biotransformation of a medication is usually unnoticed because it is usually overshadowed by the activity of hepatic enzymes. However, the increase in the expression and/or activity of a placental enzyme following its induction can be determined in vitro utilizing postpartum tissue obtained from term or preterm placentas.
Alternatively, the increase in the activity of an allosteric enzyme does not require its induction. An allosteric enzyme has a substrate and an effector binding sites. The first is the active site to which the substrate binds and is biotransformed to a metabolite. The other site (allosteric) is located at a separate part of the quaternary structure of the enzyme where a ligand (the effector molecule) binds. The binding of the effector molecule causes either an increase in the activity of the enzyme in its biotransformation of the substrate (positive cooperativity) or a decrease (negative cooperativity). To the best of our knowledge, the allosteric type of enzymes has not been documented for CYP enzymes though data on the kinetics of several of their reactions revealed atypical steady state kinetics and suggested their existence (36). Several authors hypothesized the presence of two substrates binding sites, and a separate allosteric binding site (25,37,38).
In general, an increase in the activity of an enzyme that biotransforms a medication will result in enhanced first pass-metabolism, reduced bioavailability, decreased plasma concentration, and ultimately efficacy. On the other hand, for medications that are biotransformed to more active or reactive metabolites, increase in the enzyme activity could cause adverse effects and will be further discussed in this chapter.
Enzyme Inhibition
The simultaneous administration of two medications that are substrates of the same enzyme will result in their biotransformation at lower rates. The medication with higher affinity (lower Km) will be initially/preferentially biotransformed by the enzyme but the second medication will eventually compete with the first for the same substrate-binding site of the enzyme (competitive inhibition). The result is a higher plasma concentration of the two drugs than that predicted by their PK parameters, the prolongation of the pharmacological effects, and an increase in the likelihood of side effects.
The biotransformation of medication may be also inhibited by a component present in the diet. For example, grapefruit juice inhibits activity of hepatic CYP3A4 enzyme responsible for the biotransformation of numerous medications.
On the other hand, the administered medication could be pharmacologically inactive but is biotransformed to an active metabolite by a metabolic enzyme. In this example, inhibition of the responsible enzyme decreases the efficacy of the medication (12,35).
Activation or inhibition of an enzyme that occurs due to the concomitant administration of two or more medications is often referred to as drug-drug interactions (DDI).
Factors Affecting Placental Biotransformation of Medications
The activity of placental enzymes, determined in vitro, varies widely between individuals. This variability in enzyme activity could be explained by one or more factors that include—but not limited to—fetal race, frequency of single nucleotide polymorphisms (SNPs) in the gene coding for the enzyme, maternal exposure to environmental factors, dietary components and contaminants, as well as the administration of a medication that acts as an activator or inhibitor. For example, a wide range of activity was reveled for placental microsomal CYP19 responsible for the biotransformation of methadone and buprenorphine, the opiates used for treatment of the pregnant heroin/opiate addict. The observed variability in enzyme activity was due to differences in the rates of formation of the metabolites (Vmax) rather than changes in the affinity of the substrates to the enzyme (apparent Km) (5,6,7).
Single nucleotide polymorphisms (SNP) in a gene encoding a placental enzyme may also affect the expression of its mRNA, protein, or activity. For example, in nonpregnant women, the coding sequence variants in CYP19—which is also the major placental CYP enzyme—resulted in a decrease in its activity in catalyzing the aromatization of androgens, thus decreasing estrogen levels (39). This phenotype variant of CYP19 was associated with conditions related to low estrogen levels such as the risk of osteoporosis in postmenopausal women (40) and higher breast cancer survival (41). On the other hand, other variants in CYP19 coding sequences were associated with an increase in the activity of CYP19 and higher risk of premenopausal breast cancer (39).
Gestational age is another factor that could also affect the activity of placental enzymes. For example, the activity of placental CYP19 in the biotransformation of methadone increased with gestational age (11). However, this reported increase in CYP19 activity was not based on its determination in the same placenta at sequential periods of gestation. Rather, the activity of CYP19 at a gestational age represented the mean of several individual placentas delivered within a period of 3 to 4 weeks. The interpretation of the data obtained could be misleading since the etiology of preterm birth, as well as the reasons for each preterm delivery, is often unknown. In other words, it is unclear whether the determined expression/activity of the placental enzymes at early gestational ages is a factor of age or a result of the medical condition leading to the preterm delivery.
The activity of placenta enzymes could be also affected by drug interactions. There are numerous examples of DDI involving hepatic enzymes but much less is known for those in extrahepatic tissues including the placenta. Our knowledge of DDI involving placental enzymes is limited because of their smaller contribution to the total amount of the metabolites formed in vivo by hepatic enzymes. Another type of drug interaction observed in trophoblast tissue was for an enzyme catalyzing a reaction in a placental metabolic pathway being also responsible for the biotransformation of a medication. For example, placental CYP19 is a key enzyme in the conversion of androgens to estrogens and provides approximately 50% of their concentration in the maternal circulation. In addition, CYP19 is the major placental microsomal enzyme responsible for the in vitro biotransformation of methadone and buprenorphine, the opiates used for maintenance of the pregnant opiate addict (5,7). Evidence for this type of interaction in vivo was also provided in the report indicating lower levels of estriol in plasma of pregnant women maintained on methadone (42). The in vitro inhibition of placental conversion of androgens to estrogens by methadone and buprenorphine supported the dual role of CYP19 in the biosynthesis of estrogens and the biotransformation of opiates (43). Other medications that inhibit placental CYP19 activity are aminoglutethimide, an inhibitor of adrenocortical steroid synthesis; azoles, which are used as antifungal (e.g., econazol and miconazol) or cytostatic agent (fadrozole) (44,45,46,47,48,49,50,51,52,53,54,55,56,57,58,59,60,61,62,63,64,65,66); natural substances such as components of grapefruit juice, plant flavones, α-naphthoflavone (45); and others.
The above information indicates that human placenta could be a site for drug interactions and underscores the importance of identifying the enzymes responsible for the biotransformation of medications administered during pregnancy.
Significance of Placental Biotransformation of Medications
The placenta is exposed to all compounds present in the maternal blood including medications administered during pregnancy. The extent of fetal exposure to these compounds depends on their transfer across the placenta. The major determinants for xenobiotics transfer across human placenta have been extensively reviewed by several investigators (15,47) and can be divided into three major groups: properties of the drug, functions of placental enzymes and transporters, and maternal and fetal factors/medical conditions. It should be noted here that our knowledge of the concentration of a medication in the fetal circulation is limited to that in cord blood at delivery or the newborn.
Of direct relevance to this chapter is the relation between the activity of placental enzymes in the biotransformation of a medication and the extent of fetal exposure to the drug and its metabolites. The rates and amounts of metabolite formed, for each medication, vary widely between placentas (3,4,5,6,7,8,9). Moreover, the activity of placental enzymes constitutes a fraction of that of hepatic enzymes and is often considered as insignificant. However, this smaller amounts of the metabolites formed should not negate their importance because they are in close proximity to the fetal capillaries and consequently are more accessible to the fetal circulation than those formed by maternal hepatic enzymes (9,48). Consequently, if one or more metabolites formed in the placenta is pharmacologically active or toxic (13,14), then their effects on the fetus, whether favorable or unfavorable, might be more pronounced than those formed by hepatic enzymes. In general, the placenta contributes 10% or less of the total metabolites formed for a given medication (49,50). In that respect, the placenta should be viewed as a second line of defense that protects fetus from exposure to xenobiotics, the first being maternal liver. The rationale behind this view is as follows: the weight of a newborn averages between 4% and 8% of the mother’s at delivery and the volume of the newborn circulation at term (500 mL or less) is approximately 7% to 8% of that of the pregnant woman (6 to 7 L). Therefore, the “lower” activity of the placental enzymes in the biotransformation of medications should be adequate for protecting the fetus from exposure to xenobiotics/medications in the maternal circulation.
Placental and hepatic enzymes could biotransform the same medication to structurally different metabolites as reported for 17-α-hydroxyprogesterone caproate (17-HPC), used for treatment of recurrent preterm deliveries. Human hepatic enzymes biotransform 17-HPC to several mono-, di-, and trihydroxylated metabolites. On the other hand, placental microsomal enzymes biotransforformed 17-HPC to monohydroxylated derivatives only and two of these metabolites were unique to the tissue, that is, were not formed by hepatic enzymes (10).
Table 12.1 Metabolites Formed In Vivo and In Vitro | ||||||||||||||||||||||||||||||||||||||||||||||||||||||||||||||||||||||||||||||||
---|---|---|---|---|---|---|---|---|---|---|---|---|---|---|---|---|---|---|---|---|---|---|---|---|---|---|---|---|---|---|---|---|---|---|---|---|---|---|---|---|---|---|---|---|---|---|---|---|---|---|---|---|---|---|---|---|---|---|---|---|---|---|---|---|---|---|---|---|---|---|---|---|---|---|---|---|---|---|---|---|
|
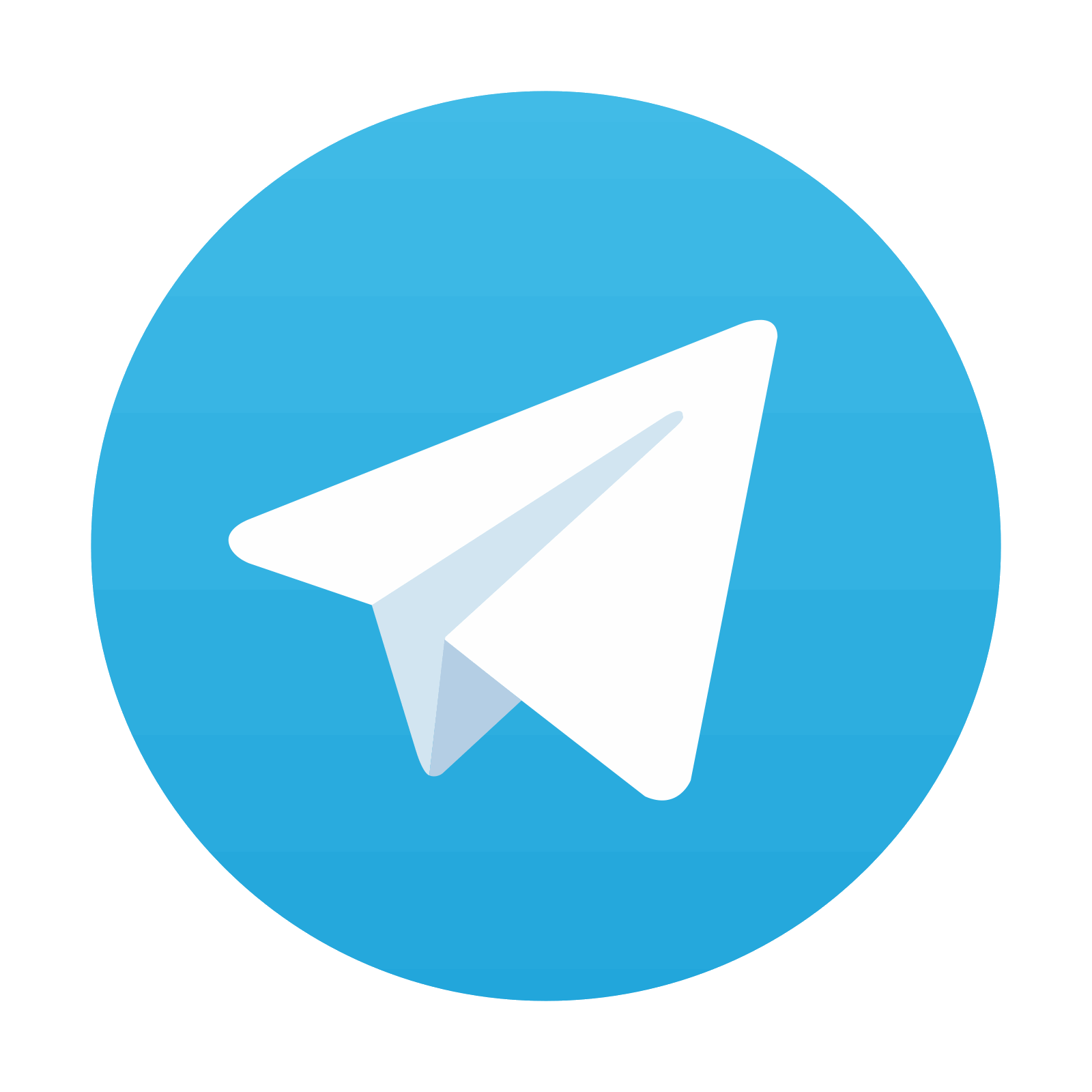
Stay updated, free articles. Join our Telegram channel
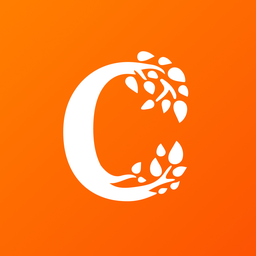
Full access? Get Clinical Tree
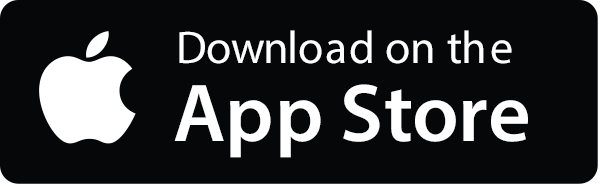
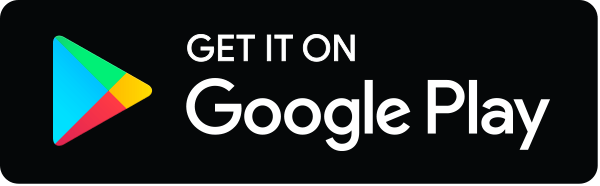