Figure 2.1 Theoretic curves for tumor control and complications as a function of radiation dose both with and without chemotherapy. TR, therapeutic range, or the difference between tumor control and complication frequency. Source: From Perez CA, Thomas PRM. Radiation therapy: Basic concepts and clinical implications. In: Sutow WW, Fernbach DJ, Vietti TJ, eds. Clinical Pediatric Oncology, 3rd ed. St. Louis, MO: Mosby; 1984:167, with permission from Elsevier.
A typical course of radiation therapy is given in 10 to 40 fractions administered over 2 to 8 weeks. Each fraction kills a fixed percentage of cells. The log cell kill model describes cell kill in very simplistic terms such that a given radiation dose will kill a certain percentage of cells and that a tumor receiving a fractionated course of radiation gets progressively smaller with time. Additionally, tumor cells may have undergone clonogenic cell death wherein they are still present but unable to reproduce. Therefore, one does not necessarily have to kill all cancer cells in order to achieve cure if the remaining ones are unable to reproduce.
The shape of the cell survival curve is best described by the linear–quadratic model with DNA as the lethal target. Radiation exerts most of its deleterious effects by causing breaks in two opposing single strands in the DNA backbone. Attempted repair of a double-stranded break may result in two possible outcomes: lethal outcomes and nonlethal outcomes. Nonlethal repair may result in a reciprocal translocation and preservation of the genetic information. In some instances, the reciprocal translocation results in the activation of an oncogene, which may manifest as a malignancy.
When a high dose of radiation (>10 Gy) is given in a single fraction, such as for stereotactic radiosurgery (SRS) or stereotactic body radiation therapy (SBRT), lethal DNA damage may not be the only resulting biologic effect. Other proposed effects include radiation-induced damage to tumor microvasculature and enhancement of antitumor immunity. These additional mechanisms of actions may explain the excellent clinical outcomes seen with SRS and SBRT.
DNA Damage Repair and the Shape of Cell Survival Curves
The concept that DNA damage repair drives the shapes of cell survival curves is well illustrated by experiments showing the effectiveness of oxygen as a sensitizer. Oxygen is known to chemically modify radiation-induced DNA damage, making it irreparable. This is known as the oxygen fixation hypothesis. Therefore, under anoxic conditions, DNA damage repair would be expected to increase considerably. Hypoxia causes the cell survival curve to shift to the right and change shape.
Biologically Equivalent Dose
Both the total dose of radiation and how fast it is delivered can produce very different outcomes. The same holds true for fractionated courses of radiation. The linear–quadratic equation can be used to derive an equation to be used as a guide to predict various biologic end points and is given by the following equation:

BED is the biologically equivalent dose, D is the total dose, d is the dose per fraction, and α/β is the dose where the alpha component of cell kill equals the beta component. These formulas are useful for conversion between different fractionation schemes: between standard and hypofractionation or between high-dose rate (HDR) and low-dose rate (LDR). This conversion is important so that doses can be compared between regimens.
The Cell Cycle
The cell cycle is an ordered set of events that results in cell growth and division into two daughter cells. The steps are G1-S-G2-M. G1 stands for “GAP 1,” and S phase stands for “synthesis” and is the point at which DNA replication occurs. Late S phase is the most radioresistant phase of the cell cycle since the DNA repair machinery for replication also can repair radiation damage. The G2 stage stands for “GAP 2,” and M phase stands for “mitosis” and is when nuclear division occurs. M phase is also the most radiosensitive phase of the cell cycle. Therefore, any DNA damage caused generally will be passed along to the daughter cells and may be fatal. This is one important reason why rapidly dividing cells such as cancers are radiosensitive. G0 is a stable state of the cell and is typically observed with well-differentiated cells that have reached the end stage of development and are no longer dividing (e.g., neuron).
INTRODUCTION TO RADIATION PHYSICS
KEY POINTS
- The most common forms of radiation used in therapy are photons (x- and γ-rays) and electrons.
- There is an increased penetration as the energy of x- or γ-rays is increased.
- X- and γ-rays differ in their source of production: x-rays are artificially produced, whereas γ-rays arise through the natural process of radioactive decay within the nucleus of an atom.
- Exposure is the amount of ionization produced in air by photons, and its unit is roentgen (R).
- Radiation deposits energy in tissue by transferring energy from photons to ionized particles, which in turn transfer energy to the medium (usually tissue).
- The SI unit for absorbed dose is also joule per kilogram or gray, which has replaced the previously used term “rad.” 1 gray = 100 cGy = 100 rads.
Radiation physics is the study of the interaction of radiation with matter. In the treatment of patients with radiation, this matter is either tumor tissue or normal tissues.
Units Used in Radiation and Radiation Therapy
Radiation is an abbreviation for electromagnetic radiation. It can occur in numerous forms. The most common forms used in radiation therapy are x-rays, γ-rays, and electrons. X- and γ-rays are also known as photons. Electrons are a form of particle beam radiation. Other particles or forms of radiation include protons, neutrons, and heavier alpha particles.
X- and γ-rays are forms of electromagnetic radiation, similar to visible light, but with a much smaller wavelength, that is, greater energy. The difference between x- and γ-rays is only their respective origins. Modern radiotherapy treatment machines termed linear accelerators or linacs create x-rays artificially by bombarding an atom or tungsten target with high-speed electrons. Linacs can produce both photon and electron beams of different energies depending on their construction. There is an increased penetration as the energy of x- or γ-rays is increased. The x-rays produced by linacs are much more penetrating than cobalt (60Co) γ-rays.
While x-rays are artificially produced, γ-rays arise through the natural process of radioactive decay within the nucleus of an atom. These radioactive isotopes can occur naturally or be created artificially. Examples include the brachytherapy sources cesium (137Cs) and iridium (192Ir) as well as cobalt (60Co) teletherapy treatment machines. Table 2.1 lists many of the more common radioactive isotopes and their physical properties. Use of radium 226 (226Ra) sources is now historic.
Table 2.1 Physical Properties and Uses of Brachytherapy Radionuclides
Electrons are negatively charged particles and will usually only penetrate a few millimeters to centimeters in tissue. Similar to photons, the higher the energy of the electron, the farther it will penetrate into the tissue, but as opposed to photons, the skin dose increases rather than decreases. Electrons are used to treat tumors or tissues close to the skin surface such as superficial inguinal nodes and tumors of the skin, including vulvar cancers.
Regarding photon interactions with tissue, the dominant process at megavoltage (MV) energies used in radiation therapy is termed the Compton effect.
Table 2.2 lists some basic units of radiation and radiation therapy, both in historic context and in terms of modern SI units. Exposure is the amount of ionization produced in air by photons, and its unit is the roentgen (R). The SI unit for exposure is coulomb per kilogram (C/kg) with 1 R = 2.58 × 10−4 C/kg air. Kerma (kinetic energy release per unit mass) defines the transfer of energy from photons to directly ionized particles. These directly ionized particles, in turn, transfer some of their energy to the medium (usually tissue). This transfer of energy is defined as the absorbed dose to the medium from the radiation beam. The SI unit for kerma is joule per kilogram (J/kg) or gray (Gy). The SI unit for absorbed dose is also joule per kilogram or gray, which has replaced the previously used term “rad.” Often the term centigray (cGy) is used. The cGy is equivalent to the rad, and 1 gray equals 100 cGy.
Table 2.2 SI Units for Radiation Therapy
RADIATION PRODUCTION
KEY POINTS
- Intensity-modulated radiation therapy (IMRT) is accomplished by using small computer-controlled leaves in the head of the machine (multileaf collimators or MLCs) to block the beam in different patterns, modulating beam intensity and creating multiple complex treatment fields. The result is improved target coverage and increased sparing of normal tissues.
- Image-guided radiation therapy (IGRT) refers to the imaging of treatment fields prior to treatment delivery in order to precisely align patient and improve treatment accuracy.
Radioactive Isotopes
As mentioned before, γ-rays are typically derived from radioactive isotopes, for example, 60Co. Electrons or beta particles also come from radioactive isotopes. Radioactivity is the result of an atom changing its “energy” state, usually to a lower “energy” state, by the emission/absorption/internal conversion of photons or electrons in the atom.
Radioactivity, or activity, is denoted by the symbol A and is defined as the number of disintegrations per unit of time. The equation describing radioactive decay is A = Aoe−λt, where Ao is the initial activity, λ is the decay constant, and t is some unit of time later. Other important units for radioactivity and radioactive decay are the half-life (T1/2) and the average life (Ta). The half-life is the amount of time necessary to reduce the original amount of material by half. T1/2 is related to the decay constant by T1/2 = 0.693/λ. Ta is related to the decay constant and the half-life by Ta = 1/λ = 1.44 T1/2.
Cesium 137 (137Cs) has replaced radium as a safer, equally effective radioisotope. It is typically used for LDR gynecologic brachytherapy in tandem and ovoid and cylinder applicators. Iridium 192 (192Ir) comes in various activities and can be used for interstitial and intracavitary gynecologic implants. It is the primary isotope for HDR applications.
Linear Accelerators
Linacs produce radiation by accelerating an initial beam of electrons across a variable electric field. This electron beam can be adjusted to control its shape and intensity before delivery to the patient. Alternatively, the electron beam can be directed to a tungsten target. The electron–target interaction creates a forward scattered photon beam or x-ray. The resulting photon beam can then be modified by the machine using filters and collimators to produce the desired radiation field shape.
Photon beams of different energies have a different absorbed dose pattern within tissues. This pattern is normally characterized as a percent depth dose or variation of dose as a function of depth within tissue. The higher-energy photons deposit dose at greater depths, and less dose is deposited at shallow depths. This is called “skin sparing” and is a characteristic of high-energy photons.
Modern linacs come equipped with MLCs and asymmetric jaws to control the shape of the radiation beam directed before it reaches the patient. MLCs are small (projected size at the patient approximately 1 cm) adjustable collimators built into the linac gantry that work together to create a shaped opening mimicking the effects of a poured block. With the advent of computer-controlled motion of the MLCs, the radiation field can be controlled to produce an IMRT treatment. In IMRT treatments, the MLCs are used to create many small fields of radiation within a larger treatment field. This adaptability allows the radiation treatment planner to create and deliver very complex treatment fields that improve target coverage while attempting to spare normal tissues.
IMRT for gynecologic cancers is currently an active subject of investigation. Published benefits of IMRT treatment plans compared to conventional three-dimensional conformal include improved dose sparing to normal tissue while simultaneously delivering higher tumor dose. One major challenge to the implementation of IMRT for gynecologic cancers has been the variation among radiation oncologists in target and normal organ delineation. To address this concern, the Radiation Therapy Oncology Group (RTOG) has defined parameters for contouring of targets and normal organs, margin size, and dose volume constraints in the postoperative setting in early cervical or endometrial cancers. An accompanying online atlas is available to improve consistency between multiple contouring physicians. The RTOG (Lim et al.) has also developed an MRI atlas for contouring the intact cervix for IMRT.
Currently, the RTOG has completed two phase II studies of postoperative IMRT to the pelvis for gynecologic cancers. RTOG 0418, which investigated postoperative pelvic IMRT alone in endometrial patients and with weekly cisplatin in cervical cancer patients, found low rates of hematologic toxicity, allowing for high rates of weekly cisplatin use. Similarly, RTOG 0921 examined postoperative IMRT with concurrent cisplatin and bevacizumab followed by carboplatin and paclitaxel for endometrial cancer; 1-year results from this phase II study show that this regimen is both feasible and well tolerated. The RTOG is currently conducting a randomized phase III study of standard versus IMRT pelvic radiation for postoperative treatment of endometrial and cervical cancer (RTOG 1203). Figure 2.2 shows a side-by-side comparison of IMRT and conventional four-field pelvic irradiation plans.
Figure 2.2 Intensity-modulated radiotherapy (IMRT) and four-field plans for (A) a large involved uterus and (B) a small uninvolved uterus. Source: From Forrest J, Presutti J, Davidson M, et al. A dosimetric planning study comparing intensity-modulated radiotherapy with four-field conformal pelvic radiotherapy for the definitive treatment of cervical carcinoma. Clin Oncol. 2012:24:e63–e70, with permission from Elsevier.
Due to the highly precise and conformal nature of IMRT delivery, organ and tissue motion is a significant concern and potential obstacle to the widespread implementation of IMRT for gynecologic cancers. A relatively new and increasingly relevant technology in radiation therapy is IGRT. Modern linacs have added on board imaging that allows the treatment fields to be recorded electronically at every treatment setup using an electronic portal imaging device. By comparing computer-generated radiographs (DRRs or digitally reconstructed radiographs) with the actual patient images, discrepancies in field shape and patient setup can be corrected before the delivered treatment. This type of corrective behavior before treatment is the foundation of IGRT. The latest variations on IGRT are the addition of computed tomography (CT) scanners or magnetic resonance imagers within the linac to verify more completely the correct alignment of the patient on the treatment table prior to treatment.
Simulation
The conventional simulator uses a diagnostic (kV) photon beam to reproduce all the gantry, collimator, and table rotations used in a linac treatment and therefore “simulates” the actual treatment. CT-based simulators (“CTSims”) have largely replaced conventional simulators and combine a diagnostic CT scanner with a software package that allows for the simulation of the necessary gantry angles and table angles modeled in the computer. Following the scan, the treatment isocenter and the full CT images are transferred to the computer planning system for further treatment planning. DRRs of the treatment field are created for later comparison to actual treatment images.
Computerized Dosimetry
In a modern radiotherapy department, computers are necessary to accurately calculate the absorbed doses to tissues. These absorbed doses within tissues are termed isodoses or lines of the same dose. To initiate this process, CT images are acquired of the area of interest at a pretreatment planning session or simulation. These scans are typically obtained on the CT simulator. Treatment targets such as pelvic lymph nodes, the uterus, or the vagina are identified through contouring on these images, as are normal tissues such as the rectosigmoid, bladder, and small bowel. Dose goals are identified for the targets and normal tissues. A dosimetrist uses this information to design the radiation treatment plan, which is reviewed by the treating physician and a physicist and is altered as needed to best address the tumor and avoid the normal organs and tissues.
The goal for most treatment plans is to treat the target to a specified dose while minimizing dose to adjacent normal tissues. Figure 2.3 illustrates the gross target volume (GTV), the clinical target volume (CTV), the planning target volume (PTV), and the treated volume. The CTV includes all of the GTV plus possible microscopic extensions. The PTV includes all of the CTV plus a margin to account for possible geometric uncertainties of the patient or treatment margin. The irradiated volume includes all of the PTV plus any margins that might be included in the treatment plan to provide minimum dose coverage to the PTV.
Figure 2.3 Schematic representation of “volumes” in radiation therapy. The treatment portal volume includes the GTV, potential areas of local and regional microscopic disease around the tumor (clinical), and a margin of surrounding normal tissue (planning). Source: From Perez CA, Purdy JA. Rationale for treatment planning in radiation therapy. In: Levitt SH, Khan FM, Potish RA, eds. Levitt and Tapley’s Technological Basis of Radiation Therapy: Practical and Clinical Applications, 2nd ed. Philadelphia, PA: Lea & Febiger; 1992. Modified in Perez CA, Brady LW, Roti JL. Overview. In: Perez CA, Brady LW, eds. Principles and Practice of Radiation Oncology, 3rd ed. Philadelphia, PA: Lippincott-Raven Publishers; 1998:1, with permission.
BRACHYTHERAPY PRINCIPLES
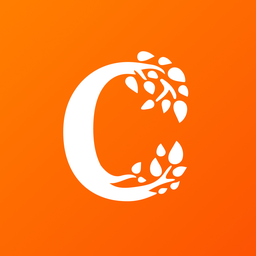
Full access? Get Clinical Tree
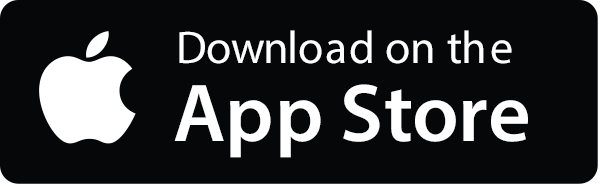
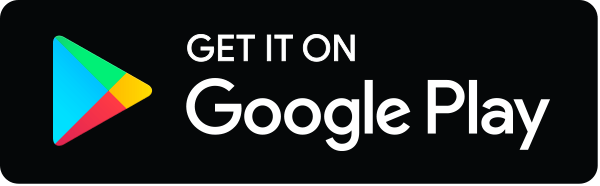