Human milk was considered a heavenly elixir, a living fluid. It was not until the end of the eighteenth century that chemical methods became available to decipher the content of milk. The biochemistry of human milk encompasses a mammoth supply of scientific data and information, most of which has been generated since 1970. Each report or study adds a tiny piece to the complex puzzle of the nutrients that make up human milk. The answers to some questions still elude us. A question as simple as the volume of milk consumed at a feeding remains a scientific challenge. The methodology must be accurate, reproducible, noninvasive, and suitable for home use night or day and must not interrupt breastfeeding. The precision analysis available for measuring the concentration of the most minuscule of elements, however, is remarkably accurate and reproducible in the laboratory. Milk has been demystified by laboratory chemistry.
Advances in analytic methods bring greater sensitivity, resolving power, and speed to the analysis of milk composition. Previously unknown and unrecognized compounds have been detected. We now know milk provides both nutrients and nonnutritive signals to the neonate. With few exceptions, all milks contain the nutrients for physical growth and development. When the offspring develops rapidly, the milk is nutrient dense; when it develops slowly, the milk is more dilute. All milks contain fat, carbohydrates, and proteins, as well as minerals, vitamins, and other nutrients. The organization of milk composition includes lipids in emulsified globules coated with a membrane, colloidal dispersions of proteins as micelles, and the remainder as a true solution. At no other time in life is a single food adequate as the sole source of nutrition.
The discussion in this chapter is limited to information perceived as immediately useful to the clinician. Considerable detail and species variability are overlooked to help focus attention on details directly influencing management. Extensive and exhaustive reviews are referenced to provide the reader with easy access to greater detail and validation of the general conclusions reported here.
Human milk is not a uniform body fluid but a secretion of the mammary gland of changing composition ( Figure 4-1 ). The first drops at the beginning of a feeding differ from the last drops. Colostrum differs from transitional and mature milks. Milk changes with the time of day and as time goes by. As concentrations of protein, fat, carbohydrates, minerals, and cells differ, physical properties such as osmolarity and pH change. The impact of changing composition on the physiology of the infant gut is beginning to be appreciated. Many constituents have dual roles, not only nutrition but infection protection, immunity, or a host of other effects.

The more than 200 constituents of milk include a tremendous array of molecules, descriptions of which continue to be refined as qualitative and quantitative laboratory techniques are perfected. Resolution of lipid chemicals has advanced dramatically in recent years, but new carbohydrates and proteins have been identified as well. Some of the compounds identified may well be intermediary products in the process that occurs within the mammary cells and may be only incidental in the final product. Milk includes true solutions, colloids, membranes, membrane-bound globules, and living cells.
Human and bovine milks are known in the greatest detail; however, much information exists about the milk of rats and mice, as well as five other species: the water buffalo, goat, sheep, horse, and pig. Several are listed in Table 4-1 . Miscellaneous data are available on the milk of 150 more species, but almost no data are available for another 4000 species. Jenness and Sloan have compiled a summary of 140 species from which a sampling has been extracted ( Table 4-2 ). The constituents of milk can be divided into the following groups, according to their specificity:
- 1.
Constituents specific to both organ and species (e.g., most proteins and lipids)
- 2.
Constituents specific to organ but not to species (e.g., lactose)
- 3.
Constituents specific to species but not to organ (e.g., albumin, some immunoglobulins)
Species | Days Required to Double Birth Weight | Content of Milk (%) | |||
---|---|---|---|---|---|
Fat | Protein | Lactose | Ash | ||
Human | 180 | 3.8 | 0.9 | 7.0 | 0.2 |
Horse | 60 | 1.9 | 2.5 | 6.2 | 0.5 |
Cow | 47 | 3.7 | 3.4 | 4.8 | 0.7 |
Reindeer | 30 | 16.9 | 11.5 | 2.8 | – |
Goat | 19 | 4.5 | 2.9 | 4.1 | 0.8 |
Sheep | 10 | 7.4 | 5.5 | 4.8 | 1.0 |
Rat | 6 | 15.0 | 12.0 | 3.0 | 2.0 |
Mammalian Species (in Taxonomic Position) | Total Solids | Fat | Casein | Whey Protein | Total Protein | Lactose | Ash |
---|---|---|---|---|---|---|---|
Human | 12.4 | 3.8 | 0.4 | 0.6 | – | 7.0 | 0.2 |
Baboon | 14.4 | 5.0 | – | – | 1.6 | 7.3 | 0.3 |
Orangutan | 11.5 | 3.5 | 1.1 | 0.4 | – | 6.0 | 0.2 |
Black bear | 44.5 | 24.5 | 8.8 | 5.7 | – | 0.4 | 1.8 |
California sea lion | 52.7 | 36.5 | – | – | 13.8 | 0.0 | 0.6 |
Black rhinoceros | 8.1 | 0.0 | 1.1 | 0.3 | – | 6.1 | 0.3 |
Spotted dolphin | 31.0 | 18.0 | – | – | 9.4 | 0.6 | – |
Domestic dog | 23.5 | 12.9 | 5.8 | 2.1 | – | 3.1 | 1.2 |
Norway rat | 21.0 | 10.3 | 6.4 | 2.0 | – | 2.6 | 1.3 |
Whitetail jackrabbit | 40.8 | 13.9 | 19.7 | 4.0 | – | 1.7 | 1.5 |
Normal Variations in Human Milk
In defining the constituents of human milk, it is important to recognize that the composition varies with the stage of lactation, the time of day, the sampling time during a given feeding, maternal nutrition, and individual variation. Many early interpretations of the content of human milk were based on spot samples or even pooled samples from multiple donors at different times and stages of lactation. Samples obtained by pumping may vary from those obtained by the suckling infant because some variation exists in content among the various methods of pumping. Banked donor milk differs from freshly expressed, mature milk in nutrient content and energy value.
Daytime consumption of milk in a given infant varies between 46% and 58% of the total 24-hour consumption, so that reliance on less than a 24-hour sampling may be misleading. Data from samples taken every 3 hours showed a variation in milk concentration of nitrogen, lactose, and fat, and in the volume of milk, by time of day ( Figure 4-2 ). Furthermore, statistically significant diurnal changes occurred in the concentration of lactose and in the volume within individual subjects, but the times of those changes were not consistent for each individual. Some individuals varied as much as two-fold in volume production from day to day. A significant difference in the concentrations of both fat and lactose and the volume of milk produced by each breast was also found. At the extreme, the less productive breast yielded only 65% of the volume of the other breast.

The variation in the fat content has received some attention. Fat content changes during a given feeding, increasing as the feeding progresses. Fat content rises from early morning to midday; as reported in early studies, when feedings were controlled the volume increased from two to five times. Multiple studies in different countries and different decades, summarized by Jackson et al., reveal that some of the variation is related to other factors. Demand feeding (mothers in 1988 in Thailand) has a different circadian variation than scheduled feeding (mothers in 1932 in the United States) ( Figure 4-3 ). In the later part of the first year of lactation, fat content diminishes. Work done by Atkinson et al. that was confirmed by other investigators showed that the nitrogen content of the milk of mothers who deliver prematurely is higher than that of those whose pregnancies reach full term. For a given volume of milk, the premature infant would receive 20% more nitrogen than the full-term infant if each were fed his or her own mother’s milk. Other constituents of milk produced by mothers who deliver prematurely have also been studied. Some milk banks now provide donor milk from mothers who have delivered prematurely.

An additional consideration in reviewing information available on the levels of various constituents of milk is the technique used to derive the data. In 1975, Hambraeus reported less protein in human milk than originally calculated (see Table 4-1 ). The present techniques of immunoassay measure the absolute amounts; earlier figures were derived from calculations based on measurements of the nitrogen content. Of the nitrogen in human milk, 25% is nonprotein nitrogen (NPN). Cow milk has only 5% NPN.
A major concern about variation in content of human milk is related to the mother’s diet. Maternal diet is of particular concern when the mother is malnourished or eats an unusually restrictive diet. Malnourished mothers have approximately the same proportions of protein, fat, and carbohydrate as well-nourished mothers, but they produce less milk. Levels of water-soluble vitamins, such as ascorbic acid, thiamin, and vitamin B 12 , are quickly affected by deficient diets. “From a nutritional perspective, infancy is a critical and vulnerable period. At no other stage in life is a single food adequate as a sole source of nutrition,” writes Picciano. This results from the immaturity of the tissues and organs involved in the metabolism of nutrients, which limits the ability to respond to nutrition excesses and deficiencies. The system is species-specific and depends on the presence of the self-contained enzymes and ligands to facilitate digestion at the proper stage while preserving function (such as secretory immunoglobulin A [sIgA]). The system continues to facilitate absorption and utilization.
Mother’s milk is recommended for all infants under ordinary circumstances, even if the mother’s diet is not perfect, according to the Committee on Nutrition During Pregnancy and Lactation of the Institute of Medicine.
The Cycles of Lactation
Two distinct phases occur during pregnancy, which have been identified as mammogenesis and lactogenesis I. Mammogenesis is the developmental differentiation that begins in early pregnancy. It includes the proliferation of the ductal tree, which results in the sprouting of multiple alveoli.
Mammogenesis results in the enlargement of the breast during pregnancy due to the proliferation of the ductoalveolar structure. Careful study of this development has been documented utilizing computerized breast measurement, first reported by Cox et al. in 1999. Computerized breast measurement is an accurate noninvasive technology that is being used to determine changes in the size of human breasts (see Chapter 8, p. 261 ). A longitudinal study using computerized breast measurement from before conception through pregnancy and lactation showed that growth began at week 10 of pregnancy. It was found that seven of eight women studied had an increase in breast size of about 170 mL with considerable individual variation on rate of change. Most women continued this growth immediately postpartum; at 1 month postpartum they had an average 211 mL of growth. The authors correlated this growth through pregnancy with an increase in placental lactogen.
Stage I lactogenesis is the onset of milk secretion and begins with the early changes in the mammary gland during pregnancy and continues until full lactation has occurred after delivery. Stage I begins when small quantities of milk components such as casein and lactose are secreted. This amount is held in check by high levels of circulating progesterone. The first milk obtained by the newborn at birth is called colostrum, and the milk produced in the first 10 days is called transitional milk. Full volume is obtained in the next stage, lactogenesis II. The terms will be used in this text for clinical purposes ( Figure 4-4 ). Neville et al. state that the terms “colostrum” and “transitional milk” do not describe the mammary secretion product during the first 4 days or from days 4 to 10 postpartum. It has always been recognized that the content changes rapidly in the first 4 days and then more slowly in the next 6 days or so as a continuum. They suggest the abandonment of these terms. Colostrum and transitional milk are convenient clinical terms that are useful descriptive terms.

Prepartum Milk
Prepartum milk is the first stage of lactogenesis and is especially conspicuous in other species, such as the goat. It provides evidence that the junctions between alveolar cells are “leaky” during pregnancy, allowing fluid and solutes to flow between the milk space and the interstitial fluid of the mammary gland. Figure 4-5 illustrates the composition of this milk in humans. The lactose concentration is directly correlated with that of potassium, but sodium and chloride are inversely related to lactose ( Tables 4-3 through 4-7 ).

Milk Component | Units | Mean ± SD ( n ) | Milk Component | Units | Mean ± SD ( n ) |
---|---|---|---|---|---|
Mean days prepartum | 20.21 ± 12.18 (11) | Calcium | mg/dL | 25.35 ± 8.48 (10) | |
Lipid | % | 2.07 ± 0.98 (11) | Magnesium | mg/dL | 5.64 ± 1.44 (10) |
Lactose | mM | 79.78 ± 21.68 (9) | Citrate | mM | 0.40 ± 0.17 (8) |
Protein | g/dL | 5.44 ± 1.71 (8) | Phosphate | mg/dL | 2.32 ± 0.70 (9) |
Glucose | mM | 0.35 ± 0.16 (8) | Ionized calcium | mM | 3.25 ± 0.84 (6) |
Sodium | mM | 61.26 ± 25.82 (10) | pH | 6.83 ± 0.18 (6) | |
Potassium | mM | 18.30 ± 5.67 (10) | Urea | mg/dL | 14.87 ± 2.40 (9) |
Chloride | mM | 62.21 ± 17.44 (10) | Creatinine | mg/dL | 1.47 ± 0.35 (9) |
* Small samples of mammary secretion were obtained three times in prepartum period from each of 11 women. In some cases, volumes were insufficient for all analyses.
Country | No. Days Measured | Sex | Month of Lactation | |||||||||||
---|---|---|---|---|---|---|---|---|---|---|---|---|---|---|
< 1 | 1-2 | 2-3 | 3-4 | 4-5 | 5-6 | |||||||||
n | mL/24 h | n | mL/24 h | n | mL/24 h | n | mL/24 h | n | mL/24 h | n | mL/24 h | |||
U.S. | 2 | M, F | – | – | 3 | 691 | 5 | 655 | 3 | 750 | – | – | – | – |
U.S. | 1-2 | M, F | 46 | 681 | – | – | – | – | – | – | – | – | – | – |
Canada | ? | M, F | – | – | – | – | – | – | 33 | 793 | 31 | 856 | 28 | 925 |
Sweden | ? | M, F | 15 | 558 | 11 | 724 | 12 | 752 | – | – | – | – | – | – |
U.S. | 3 | M, F | – | – | 11 | 600 | – | – | 2 | 833 | – | – | 3 | 682 |
U.S. | 3 | M, F | – | – | 26 | 606 | 26 | 601 | 20 | 626 | – | – | – | – |
U.K. | 4 | M | – | – | 27 | 791 | 23 | 820 | 18 | 829 | 5 | 790 | 1 | 922 |
F | – | – | 20 | 677 | 17 | 742 | 14 | 775 | 6 | 814 | 4 | 838 | ||
U.S. | 1 | M, F | 16 | 673 ± 192 SD | 19 | 756 ± 170 | 16 | 782 ± 172 | 13 | 810 ± 142 | 11 | 805 ± 117 | 11 | 896 ± 122 |
Month of Lactation | ||||||||
---|---|---|---|---|---|---|---|---|
7 | 8 | 9 | 10 | 11 | 12 | |||
U.S. | 1 | M, F | 875 ± 142 SD | 834 ± 99 | 774 ± 180 | 691 ± 233 | 516 ± 215 | 759 ± 28 |
Day Postpartum | |||||||
---|---|---|---|---|---|---|---|
Component | 1 | 2 | 3 | 4 | 5 | 14 | 28 |
Yield (g/24 h) | 50 | 190 | 400 | 625 | 700 | 1100 | 1250 |
Lactose (g/L) | 20 | 25 | 31 | 32 | 33 | 35 | 35 |
Fat (g/L) | 12 | 15 | 20 | 25 | 24 | 23 | 29 |
Protein (g/L) | 32 | 17 | 12 | 11 | 11 | 8 | 9 |
Day | Component (g/dL) | ||
---|---|---|---|
Total Protein | Lactose | Triacylglycerols | |
1 | 2.95 ± 0.86 | 4.07 ± 0.98 | 2.14 ± 0.86 |
3 | 1.99 ± 0.22 | 4.98 ± 0.76 | 3.01 ± 0.77 |
5 | 1.82 ± 0.21 | 5.13 ± 0.54 | 3.06 ± 0.45 |
8 | 1.73 ± 0.27 | 5.38 ± 0.97 | 3.73 ± 0.70 |
15 | 1.56 ± 0.42 | 5.42 ± 0.76 | 3.59 ± 0.86 |
22 | 1.51 ± 0.27 | 5.34 ± 0.96 | 3.87 ± 0.68 |
29 | 1.5 ± 0.27 | 4.01 ± 1.13 | 4.01 ± 1.13 |
36 | 1.4 ± 0.26 | 5.34 ± 1.31 | 4.01 ± 1.20 |
Measurement | Prepartum | Postpartum | |||
---|---|---|---|---|---|
Early | Late | Colostrum | Transitional | Mature | |
Fat (%) | – | 2 | 2 | 2.9 | 3.6 |
Fat (g) | – | – | 2.9 | 3.6 | 3.8 |
Lipid (g/dL) | 1.15 | 1.28 | 3.16 | 3.49 | 4.14 |
Phospholipid (mg/dL) | 37 | 40 | 35 | 31 | 27 |
Percentage of total lipid | 3.2 | 3.1 | 1.1 | 0.9 | 0.6 |
Cholesterol (mg/dL) | – | – | 29 | 20 | 13.5 |
Colostrum
The stages in the continuum of human milk in traditional nomenclature are colostrum, transitional milk, and mature milk, and their relative contents are significant for newborns and their physiologic adaption to extrauterine life.
The mammary secretion during the first few days consists of a yellowish, thick fluid: colostrum. The residual mixture of materials present in the mammary glands and ducts at delivery and immediately after is progressively mixed with newly secreted milk, forming colostrum. Human colostrum is known to differ from mature milk in composition, both in the nature of its components and in the relative proportions of these components. The first changes are in sodium and chloride concentrations and an increase in lactose, probably as a result of the closure of the tight junctions. The specific gravity of colostrum is 1.040 to 1.060. The mean energy value is 67 kcal/dL compared with 75 kcal/dL of mature milk. The volume varies between 2 and 20 mL per feeding in the first 3 days. The total volume per day also depends on the number of feedings and is reported to average 100 mL in the first 24 hours (which is different from the first day, depending on the time of delivery) (see Table 4-4 ). Tables 4-5 and 4-6 list the yield and composition of colostrum (1 to 5 days) and mature milk (14 days and beyond). The increased production of citrate is paralleled by the increase in volume ( Figures 4-6 and 4-7 ). The result is a decrease in sodium and chloride and an increase in lactose concentration due to water dilution. ,


The antepartum milk glucose level is 0.35 ± 0.16 mmol/L (see Table 4-3 ). Glucose levels vary among individuals. Glucose decreases during a feed as aqueous phase decreases and lipid increases. In early colostrum, glucose passes into the milk via the paracellular pathway and parallels lactose. When lactation is fully established, glucose levels are unrelated to lactose levels. In mature milk, the level is 1.5 ± 0.4 mmol/L.
Dewey et al. clearly demonstrate that in a well-established milk supply, volume depends on infant demand, and the residual milk available at each feeding is comparable in both low-intake and average-intake dyads. Infant birth weight, weight at 3 months, and total time nursing were positively associated with intake. The volume also varies with the mother’s parity. Women who had other pregnancies, particularly those who previously nursed infants, have colostrum more readily available at delivery, and the volume increases more rapidly.
The yellow color of colostrum results from β-carotene. The ash content is high, and the concentrations of sodium, potassium, and chloride are greater than those of mature milk. Protein, fat-soluble vitamins, and minerals are present in greater percentages than in transitional or mature milk. sIgA and lactoferrin increase in concentration. The complex sugars, oligosaccharides, also increase, adding to the infection protection properties at this stage. It has been suggested that the mammary gland is actually evolved in part as an inflammatory response to tissue damage and infection and that the nutritional function then followed the protective function.
The higher protein, lower fat, and lactose solution is rich in immunoglobulins, especially sIgA. The number of immunologically competent mononuclear cells is at its highest level. Fat, contained mainly in the core of the fat globules, increases from 2% in colostrum to 2.9% in transitional milk and to 3.6% in mature milk. The concentration of fat in the prepartum secretion is only 1 g/dL, and the distribution among classes of lipids differs. Prepartum milk is 93% triglycerides, increasing to 97% in colostrum, with diglycerides, monoglycerides, and free fatty acids all increasing from prepartum to postpartum secretions. Phospholipid levels decline during the same period. Prepartum secretions contain higher amounts of membrane components such as phospholipids, cholesterol, and cholesteryl esters, which decline from colostrum to mature milk.
Cholesterol appears to be synthesized in the mammary gland. Beyond its use in brain tissue development, the myelinization of nerves, and as the base of many enzymes, the role of cholesterol in colostrum remains elusive. Little research has been done on cholesterol in colostrum.
Colostrum facilitates the establishment of Lactobacillus bifidus flora in the digestive tract. Colostrum also facilitates the passage of meconium. Meconium contains an essential growth factor for L. bifidus and is the first culture medium in the sterile intestinal lumen of the newborn infant. Human colostrum is rich in antibodies, which may provide protection against the bacteria and viruses that are present in the birth canal and associated with other human contact. Colostrum also contains antioxidants, which may function as traps for neutrophil-generated reactive oxygen metabolites.
The progressive changes in mammary secretion in both breastfeeding and nonbreastfeeding women between 28 and 110 days before delivery and up to 5 months after delivery were followed by Kulski and Hartmann to study the initiation of lactation. During late pregnancy the secretion contained higher concentrations of proteins and lower concentrations of lactose, glucose, and urea than those contained in milk secreted when lactation was well established. The concentrations of sodium, chloride, and magnesium were higher and those of potassium and calcium lower in colostrum than in milk. The osmolarity was relatively constant throughout the study. The authors described a two-phase development of lactation, with an initial phase of limited secretion in late pregnancy and a true induction of lactation in the second phase, 32 to 40 hours postpartum. Comparison with the nonlactating women revealed similar secretion during the first 3 days postpartum. This, however, was abruptly reversed during the next 6 days as mammary involution progressed. Obtaining samples in these women, however, may have served to prolong the period of production. The authors point out that although breastfeeding was not necessary for the initiation of lactation in this study, it was essential for the continuation of lactation.
The yield of milk has been calculated from absolute values to demonstrate the increase in output of milk constituents during lactogenesis ( Figure 4-8 ). Dramatic increases occurred in the production of all the milk constituents. The components synthesized by the mammary epithelium (lactose, lactalbumin, and lactoferrin) increased at a rate greater than those for IgA or proteins derived from the serum IgG and IgM. The greatest difference in yield between day 1 and day 7 postpartum was for glucose.

A survey of the fatty acid components shows the lauric acid and myristic acid contents to be low in concentration the first few days. When the lauric and myristic acids increased, C 18 acids decreased. Palmitoleic acid increased at the same rate as the myristic acid. From this, it was concluded that the early fatty acids are derived from extramammary sources, but the breast quickly begins to synthesize fatty acids for the production of transitional and mature milk (see Table 4-7 ). The total fat content may have a predictive value. It was shown that 90% of the women whose milk contained 20 g or more of fat per feeding on the seventh day were successfully breastfeeding 3 months later. Women who had only 5 to 10 g of fat on the seventh day had an 80% dropout rate by 3 months.
Colostrum’s high protein and low fat are in keeping with the needs and reserves of the newborn at birth. Although the content of total nitrogen or any amino acid in breast milk in 24 hours is grossly related to the volume produced, the concentration in milligrams per deciliter (mg/dL) is not so related. The relative distribution of the individual amino acids in each deciliter (100 mL) of milk differs in each mother. The colostrum may actually reflect a transitional maternal blood picture, which is associated with nitrogen metabolism of the postpartum period. The postpartum period is one of involution of body tissue and catabolism of protein in the mother ( Figure 4-9 ).

Colostrum contains at least two separate antioxidants, an ascorbate-like substance and uric acid. These antioxidants may function in the colostrum as traps for neutrophil-generated, reactive oxygen metabolites. The aqueous human colostrum interferes with the oxygen metabolic and enzymatic activities of the polymorphonuclear leukocytes that are important in the reaction to acute inflammation. This supports the belief that human milk is antiinflammatory.
The mineral and vitamin reserves of the newborn infant are related to the maternal diet. A fetal supply of vitamin C, iron, and amino acids is adequate because infant blood levels exceed those of the mother. Colostrum is rich in fat-soluble vitamin A, carotenoids, and vitamin E. The average vitamin A level on the third day can be three times that of mature milk. Similarly, carotenoids in colostrum may be 10 times the level in mature milk, and vitamin E may be two to three times greater than in mature milk.
Studies that looked at multiparas versus primiparas showed that the volume of milk was significantly greater on day 5 with earlier appearance of the casein band in multiparas ( Figure 4-10 ).

Sodium as a Predictor of Successful Lactogenesis
Early in lactogenesis, the sodium levels are high but quickly drop from 60 to 20 mmol by day 3 in women who have been fully feeding their infant. Observations by Morton have shown that high breast milk sodium concentrations on day 3 are suggestive of impending lactation failure. Even women who remove only a small amount of milk daily for research purposes have the physiologic drop in sodium.
Lactogenesis Stage II
Even though best practices recommend breastfeeding shortly after birth and frequently thereafter, Kulski et al. showed that milk removal is not needed for the programmed physiologic changes in mammary epithelium to trigger lactogenesis II. Studies by Woolridge confirmed this when no effect of breastfeeding in the first 24 hours was observed on later milk transfer to the infants. However, time of first breastfeeding and frequency of breastfeeding on day 2 are correlated with milk volume by day 5. No relationship between concentration of prolactin in the plasma and the rate of milk synthesis in either the short or long term was found by Cox et al.
Transitional Milk
The milk produced between the colostrum and mature milk stages is transitional milk; its content gradually changes. The transitional phase is approximately from 7 to 10 days postpartum to 2 weeks postpartum. The concentration of immunoglobulins and total protein decreases, whereas the lactose, fat, and total caloric content increases. The water-soluble vitamins increase, and the fat-soluble vitamins decrease to the levels of mature milk.
In a study of transitional milks, breast milk samples were obtained from healthy mothers of term infants on the 1st, 3rd, 5th, 8th, 15th, 22nd, 29th, and 36th days of lactation by Hibberd et al., who defined the first day of lactation to be the 3rd day postpartum. The researchers pooled 24-hour samples for analysis, and the remainder was fed to the baby. The authors found a high degree of variability, not only among mothers but also within samples from the same mother. The maximum value in almost every case was more than twice the minimum. They were able to show, however, that the changes in composition were rapid before day 8, and then progressively less change took place until the composition was relatively stable before day 36 (see Tables 4-5 and 4-6 ).
Mature Milk
Water
In almost all mammalian milks, water is the constituent in the largest quantity, with the exception of the milk of some arctic and aquatic species, who produce milks with high-fat content (e.g., the northern fur seal produces milk with 54% fat and 65% total solids) (see Table 4-2 ). All other constituents are dissolved, dispersed, or suspended in water. Water contributes to the temperature-regulating mechanism of the newborn because 25% of heat loss is from evaporation of water from the lungs and skin. The lactating woman has a greatly increased obligatory water intake. If water intake is restricted during lactation, other water losses through urine and insensible loss are decreased before water for lactation is diminished. Because lactose is the regulating factor in the amount of milk produced, the secretion of water into milk is partially regulated by lactose synthesis. Investigations by Almroth show that the water requirement of infants in a hot, humid climate can be provided entirely by the water in human milk.
Human milk is a complex fluid that scientists have studied by separating the several phases by physical forces. These forces include settling; short-term, low-speed centrifugation; high-speed centrifugation; and precipitation by micelle-destroying treatments, such as using the enzyme rennin (chymosin) or reducing the pH. On settling, the cream floats to the top, forming a layer of fat (about 4% by volume in human milk) ( Table 4-8 ). Lipid-soluble components such as cholesterol and phospholipid remain with the fat. With a slow-speed spin, cellular components form a pellet. The high-speed spin brings the casein micelles into a separate phase or forms a pellet. On top of the protein pellet is a loose pellet referred to as the “fluff,” composed of membranes. , Casein precipitation (0.2% by weight) is caused by acid destruction of the micelles. The aqueous phase is whey, which also contains milk sugar, milk proteins, IgA, and the monovalent ions.
Nutrient | Amount in Human Milk ∗ (g/L ± SD) | Nutrient | Amount in Human Milk ∗ (mcg ± SD) |
---|---|---|---|
Lactose | 72.0 ± 2.5 | Calcium | 280 ± 26 |
Protein | 10.5 ± 2.0 | Phosphorus | 140 ± 22 |
Fat | 39.0 ± 4.0 | Magnesium | 35 ± 2 |
Sodium | 180 ± 40 | ||
Potassium | 525 ± 35 | ||
Chloride | 420 ± 60 | ||
Iron | 0.3 ± 0.1 | ||
Zinc | 1.2 ± 0.2 | ||
Copper | 0.25 ± 0.03 | ||
Vitamin E | 2.3 ± 1.0 | ||
Vitamin C | 40 ± 10 | ||
Thiamin | 0.210 ± 0.035 | ||
Riboflavin | 0.350 ± 0.025 | ||
Niacin | 1.500 ± 0.200 | ||
Vitamin B 6 | 93 ± 8 ¶ | ||
Pantothenic acid | 1.800 ± 0.200 | ||
Vitamin A, RE | 670 ± 200 (2230 IU) | ||
Vitamin D | 0.55 ± 0.10 | ||
Vitamin K | 2.1 ± 0.1 | ||
Folate | 85 ± 37 † | ||
Vitamin B 12 | 0.97 ‡ , § | ||
Biotin | 4 ± 1 | ||
Iodine | 110 ± 40 | ||
Selenium | 20 ± 5 | ||
Manganese | 6 ± 2 | ||
Fluoride | 16 ± 5 | ||
Chromium | 50 ± 5 | ||
Molybdenum | NR |
* Data from Pediatric nutrition handbook , ed 2, Elk Grove Village, Ill., 1985, AAP, p 363, unless otherwise indicated. Values are representative of amounts of nutrients present in human milk; some may differ slightly from those reported by investigators cited in text.
† From Brown CM, Smith CM, Picciano MF: Forms of human milk folacin and variation patterns, J Pediatr Gastroenterol Nutr 5:278, 1986.
‡ From Sandberg DP, Begley JA, Hall CA: The content, binding, and forms of vitamin B 12 in milk, Am J Clin Nutr 34:1717, 1981.
§ Standard deviation not reported; range 0.33 to 3.20.
¶ From Styslinger L, Kirksey A: Effects of different levels of vitamin B 6 supplementation on vitamin B 6 concentrations in human milk and vitamin B 6 intakes of breastfed infants, Am J Clin Nutr 41:21, 1985.
Lipids
Intense interest in the lipids in human milk has been sparked by the reports from long-range studies of breastfed infants that show more advanced development at 1 year, 8 to 10 years, and now 18 years of age compared with formula-fed infants. This attention has resulted from the interest in supplementing formula with various missing factors, such as cholesterol and docosahexaenoic acid (DHA). These compounds function in a milieu of arachidonic acid, lipases, and other enzymes, and no evidence indicates that they are effective in isolation or that more is better. The value of supplementing the mother’s diet in pregnancy and lactation is an equally important question, because dietary DHA levels have declined in the last half century as women have reduced eggs and animal organs in their diet (see Chapter 9 ).
Lipids are a chemically heterogeneous group of substances that are insoluble in water and soluble in nonpolar solvents. Lipids are separated into many classes and thousands of subclasses. The main constituents of human milk are triacylglycerols, phospholipids, and their component fatty acids, the sterols. Jensen, a renowned milk lipidologist, and his coauthors remind readers, in a comprehensive review of the lipids in human milk, of the nomenclature, such as palmitic acid (16:0), oleic acid (18:1), and linoleic acid (18:2). The figure to the left of the colon is the number of carbons and to the right is the number of double bonds. Polyunsaturated fatty acids (PUFAs) have a designation for the location of the double bond; in human milk, the designation is cis (c) , which identifies the geometric isomer. Because milk is an exceptionally complex fluid, Jensen , and other scientists have found it helpful to classify components according to their size and concentration, with solubility in milk, or lack thereof, as additional categories ( Table 4-9 ). The lipids fulfill a host of essential functions in growth and development, provide a well-tolerated energy source, serve as carriers of messages to the infant, and provide physiologic interactions, including the following:
- 1.
Allow maximum intestinal absorption of fatty acids
- 2.
Contribute about 50% of calories
- 3.
Provide essential fatty acids (EFAs) and PUFAs
- 4.
Provide cholesterol
Compartment | Major Constituents | ||
---|---|---|---|
Description | Content (%) | Name | Content (%) |
Aqueous phase | 87.0 | Compounds of Ca, Mg, PO 4 , Na, K, Cl, CO 2 , citrate, casein | 0.2 as ash |
True solution (1 nm) | |||
Whey proteins (3-9 nm) | Whey proteins: α-lactalbumin, lactoferrin, IgA, lysozyme, serum albumin | 0.6 | |
Lactose and oligosaccharides; 7.0% and 1.0% | 8.0 | ||
Nonprotein nitrogen compounds: glucosamine, urea, amino acids; 20% of total N | 35-50 mg N | ||
Miscellaneous: B vitamins, ascorbic acid | |||
Colloidal dispersion (11-55 nm, 10 16 mL − 1 ) | 0.3 | Caseins: beta and kappa, Ca, PO 4 | 0.2-0.3 |
Emulsion | |||
Fat globules (4 μ m, 1.1 10 mL − 1 ) | 4.0 | Fat globules: triacylglycerols, sterol esters | 4.0 |
Fat-globule membrane interfacial layer | 2.0 | Milk-fat-globule membrane: proteins, phospholipids, cholesterol, enzymes, trace minerals, fat-soluble vitamins | 2% of total lipid |
Cells (8-40 μ m, 10 4 -10 5 mL − 1 ) | Macrophages, neutrophils, lymphocytes, epithelial cells |
By percentage of concentration, the second greatest constituent in milk is the lipid fraction. Milk lipids provide the major fraction of kilocalories in human milk. Lipids average 3% to 5% of human milk and occur as globules emulsified in the aqueous phase. The core or nonpolar lipids, such as triacylglycerols and cholesterol esters, are coated with bipolar materials, phospholipids, proteins, cholesterol, and enzymes. This loose layer is called the milk-lipid-globule membrane, which keeps the globules from coalescing and thus acts as an emulsion stabilizer. Globules are 1 to 10 mm in diameter, with 1-mm globules predominating.
Fats are also the most variable constituents in human milk, varying in concentration over a feeding, from breast to breast, over a day’s time, overtime itself, and among individuals ( Table 4-10 ). This information is significant when testing milk samples for energy intake, fat-soluble constituents, and physiologic variation and when clinically managing lactation problems. Much of the early work was based on lactation in women who “nursed by the clock” rather than tuned into infant needs. When circadian variation in fat content was studied in a rural Thai population who had practiced demand feeding for centuries, Jackson et al. found fat concentrations in feeds in the afternoon and evening (1600 to 2000 hours) were higher than those during the night (400 to 800 hours). When Kent et al. reexamined volume, frequency of breastfeedings, and the fat content of the milk throughout the day in 71 mother-infant dyads, they found similar trends. They found, however, that fat content was 41.1 ± 7.8 g/L and ranged from 22.3 to 61.6 g/L. It was not related to time after birth or number of breastfeedings during the day. No effect on the average milk fat content was related to the sex of the infant, clustered breastfeedings, or whether the infant fed at night. Fat content was higher during the day and evening compared with night and early morning. They recommended that infants be fed on demand day and night and not by schedule.
Factor | Influence |
---|---|
Duration of gestation | Shortened gestation increases the long-chain polyunsaturated fatty acids secreted |
Stage of lactation (↑) | Phospholipid and cholesterol contents are highest in early lactation |
Parity (↓) | High parity is associated with reduced endogenous fatty acid synthesis |
Volume (↓) | High volume is associated with low milk fat content |
Feeding (↑) | Human milk fat content progressively increases during a single nursing |
Maternal diet | A diet low in fat increases endogenous synthesis of medium-chain fatty acids (C6 to C10) |
Maternal energy status (↑) | A high weight gain in pregnancy is associated with increased milk fat |
When milk of the mothers of preterm infants was measured (6.6% ± 2.8%), the fat content was significantly higher in the evening (7.9% ± 2.9%) than in the morning ( D < 0.001).
It is speculated that the altered posture at night, horizontal and relatively inactive, may redistribute fat. The larger the milk consumption at a feed, the greater is the increase in fat from beginning to end of the feed. Less fat change occurs during “sleep” feeds than in the daytime. Unless 24-hour samples are collected by standardized sampling techniques, results will vary. During the course of a feeding, the fluid phase within the gland is mixed with fat droplets in increasing concentration. The fat droplets are released when the smooth muscle contracts in response to the let-down reflex.
The lipid fraction of the milk is extractable by suitable solvents and may require more than one technique to extract all the lipids. , Complete extraction in human milk is difficult because the lipids are bound to protein. From 30% to 55% of the kilocalories are derived from fats; this represents a concentration of 3.5 to 4.5 g/dL. Milk fat is dispersed in the form of droplets or globules maintained in solution by an absorbed layer or membrane. The protective membrane of the fat globules is made up of phospholipid complexes. The rest of the phospholipids found in human milk are dispersed in the skim milk fraction. Vitamin A esters, vitamin D, vitamin K, alkyl glyceryl ethers, and glyceryl ether diesters are also in the lipid fraction but do not fall into the classes listed.
Renewed interest in defining the constituents of human milk lipid has developed as investigators look for the causes of obesity, atherosclerosis, and other degenerative diseases and their relationship to infant nutrition. A number of reports of historic value have technical problems of sampling. Because the fat content of a feeding varies with time, spot samples give spurious results. Ferris and Jensen have reviewed the literature exhaustively and describe the fractionated lipid constituents in detail.
Most studies on fat content of milk have been based on a geographically limited population. Milk fat changes with diet and maternal adipose stores; Yuhas et al. studied milk samples from nine countries (Australia, Canada, Chile, China, Japan, Mexico, Philippines, the United Kingdom, and the United States). Saturated fatty acids were constant across countries, and monounsaturated fatty acids varied minimally. Arachidonic acid (C20: 4 n -6) was also similar. DHA (22: 6 n -3), however, was variable everywhere, but was dramatically different with milk from Japan having the highest values and the United States and Canada having the lowest values. Of note is the fact that the timing of collections was comparable in all countries. All samples were collected by electric pump, except in Japan where they were hand expressed.
The average fat content of pooled 24-hour samples has been reported from multiple sources to vary in mature milk from 2.10% to 5.0% ( Table 4-11 ). Maternal diet affects the constituents of the lipids but not the total amount of fat. A minimal increase in total lipid content was observed when an extra 1000 kcal of corn oil was fed to lactating mothers. A diet rich in polyunsaturated fats will cause an increased percentage of polyunsaturated fats in the milk without altering the total fat content. When the mother is calorie deficient, depot fats are mobilized, and milk resembles depot fat. When excessive nonfat kilocalories are fed, levels of saturated fatty acids increase as lipids are synthesized from tissue stores.
Lipid Class | Percentage of Total Lipids at Lactation Day | |||||
---|---|---|---|---|---|---|
3 | 7 | 21 | 42 | 84 | Immediate Extraction | |
Total lipid, % in milk ∗ | 2.04 ± 1.32 | 2.89 ± 0.31 | 3.45 ± 0.37 | 3.19 ± 0.43 | 4.87 ± 0.62 | |
Phospholipid | 1.1 | 0.8 | 0.8 | 0.6 | 0.6 | 0.81 |
Monoacylglycerol | – | – | – | – | – | ND |
Free fatty acids | – | – | – | – | – | 0.08 |
Cholesterol (mg/dL) † | 1.3 (34.5) | 0.7 (20.2) | 0.5 (17.3) | 0.5 (17.3) | 0.4 (19.5) | 0.34 |
1,2-Diacylglycerol | – | – | – | – | – | 0.01 |
1,3-Diacylglycerol | – | – | – | – | – | ND |
Triacylglycerol | 97.6 | 98.5 | 98.7 | 98.9 | 99.0 | 98.76 |
Cholesterol esters (mg) ‡ | ||||||
Number of women | 39 | 41 | 25 | 18 | 8 | 6 |
† Total cholesterol content ranges from 10 to 20 mg/dL after 21 days in most milks.
‡ Not reported, but in Bitman et al. (Bitman J, Wood DL, Mehta NR, et al: Comparison of the cholesteryl ester composition of human milk from preterm and term mothers, J Pediatr Gastroenterol Nutr 5:780, 1986), it was 5 mg/dL at 3 days and 1 mg/dL at 21 days and thereafter.
When fish oil supplementation is given during pregnancy it significantly alters the early postpartum breast milk fatty acid composition (omega-3 PUFA). Levels of omega-3 fatty acids are increased as are IgA and many other immunomodulatory factors (CD14).
A 2-week crossover study of three nursing women was done by Harzer et al., alternating high fat/low carbohydrate and the reverse. The first diet was 50% fat, 15% protein, and 35% carbohydrate for a total of 2500 calories, which resulted in a reduction of triglycerides (4.1% to 2.6%) and an increase in lactose (5.2% to 6.4%) ( Table 4-12 ).
Milk Component | Maternal Ad Lib Diet (P/S 0.53) (mg/100 g fat) | Low-Cholesterol/High-Phytosterol Diet (P/S 1.8) (mg/100 g fat) | High-Cholesterol/Low-Phytosterol Diet (P/S 0.12) (mg/100 g fat) |
---|---|---|---|
Cholesterol | 240 ± 40 | 250 ± 10 | 250 ± 20 |
Phytosterol | 17 ± 3 | 220 ± 30 | 70 ± 10 |
Dietary cholesterol | 450 ± 30 | 130 ± 5 | 460 ± 90 |
Dietary phytosterol | 23 ± 8 | 790 ± 17 | 80 ± 1 |
Total fat (%) | 3.58 ± 0.56 | 2.69 ± 0.17 | 2.66 ± 0.16 |
The U.S. Department of Agriculture (USDA) has reported that the average American diet now includes 156 g of fat per day, up from 141 g in 1947. The significant change is from animal to vegetable fat, which is now 39% of total dietary fats, especially resulting from the switch from butter and lard. A change in fatty acid content to more long-chain fatty acids and a two-fold to three-fold increase in linoleic acid have occurred. Except for 18:2 content in mature milk, the fatty acid composition is remarkably uniform unless the maternal diet is unusually bizarre.
Polyunsaturated fats include C18:2 and C18:3, or linoleic and linolenic acid. The bovine ratio of polyunsaturated to saturated fats (P/S ratio) is 4. The P/S ratio has shifted as a result of recent dietary changes to 1.3 from 1.35 in human milk. The P/S ratio is significant in facilitating calcium and fat absorption. Calcium absorption is depressed by a 4:5 P/S ratio. The breast can dehydrogenate saturated and monounsaturated fatty acids in milk synthesis.
At least 167 fatty acids have been identified in human milk; possibly others are present in trace amounts. Bovine milk has 437 identified fatty acids. Major dietary changes would greatly change fatty acid composition.
Milk from vegetarians (lacto-ovo) contained a lower proportion of fatty acids derived from animal fat and a higher proportion of PUFAs derived from dietary vegetable fat. Women who consumed 35 g or more of animal fat per day had higher C10:0, C12:0, and C18:3 but lower levels of C16:0 and C18:0. Finley et al. suggest that a maximum amount of C16:0 and C18:0 can be taken up from the blood and subsequently secreted into milk ( Table 4-13 ).
Lipid (%)/Fatty Acid | Vegetarian ∗ | Control ∗ | Vegan′ | Vegetarian † | Omnivore † |
---|---|---|---|---|---|
Number | 12 | 7 | 19 | 5 | 21 |
Saturates | |||||
6:0 | – | – | – | – | – |
8:0 | 0.16 ± 0.03 | 0.22 ± 0.01 | – | – | – |
10:0 | 1.56 ± 0.13 | 1.57 ± 0.09 | 1.8 ± 0.40 | 1.3 ± 0.51 | 0.4 ± 0.23 |
12:0 | 7.07 ± 0.78 | 5.47 ± 0.66 | 6.6 ± 0.54 | 3.2 ± 0.49 | 1.7 ± 0.35 |
14:0 | 8.16 ± 1.00 | 6.54 ± 0.73 | 6.9 ± 0.58 | 5.2 ± 0.50 | 4.5 ± 0.35 |
16:0 | 15.31 ± 0.73 | 20.48 ± 0.64 | 18.1 ± 1.34 | 21.2 ± 1.07 | 25.1 ± 0.78 |
18:0 | 4.48 ± 0.37 | 8.14 ± 0.55 | 4.9 ± 0.36 | 7.4 ± 0.35 | 9.7 ± 0.68 |
20:0 | 0.54 ± 0.02 | 0.57 ± 0.03 | – | – | – |
Total | 37.28 | 42.99 | |||
Monounsaturates | |||||
16:1 | 1.66 ± 0.14 | 3.35 ± 0.28 | 4.9 ± 0.24 | 2.9 ± 0.37 | 3.4 ± 0.35 |
18:1 | 26.89 ± 1.47 | 34.7 ± 0.86 | 32.2 ± 1.06 | 35.3 ± 1.94 | 38.7 ± 1.27 |
Total | 28.55 | 38.06 | 37.10 | 38.2 | 42.1 |
Polyunsaturates | |||||
n -6 Series | |||||
18:2 | 28.82 ± 1.39 | 14.47 ± 1.98 | 23.8 ± 1.40 | 19.5 ± 3.62 | 10.9 ± 0.96 |
20:2 | 0.72 ± 0.03 | 0.50 ± 0.03 | – | – | – |
20:3 | 0.62 ± 0.03 | 0.56 ± 0.03 | 0.44 ± 0.03 | 0.42 ± 0.07 | 0.40 ± 0.08 |
20:4 | 0.68 ± 0.03 | 0.68 ± 0.03 | 0.32 ± 0.02 | 0.38 ± 0.05 | 0.35 ± 0.03 |
Total | 30.84 | 16.21 | 31.4 | 27.5 | 18.4 |
n -3 Series | |||||
18:3 | 2.76 ± 0.16 | 1.85 ± 0.16 | 1.36 ± 0.18 | 1.25 ± 0.22 | 0.49 ± 0.06 |
22:6 | 0.22 ± 0.08 | 0.27 ± 0.08 | 0.14 ± 0.06 | 0.30 ± 0.05 | 0.36 ± 0.07 |
Total | 3.05 | 2.12 | 1.50 | 1.55 | 0.86 |
Dietary information | |||||
Vegetarian (col. 2): whole cereal grains, 50-60%; soup, 5%; vegetables, 20-25%; beans and sea vegetables, 5-10%; macrobiotic diet for a mean of 81 months; no meat or dairy products; occasional seafood, nuts, and fruit | Vegan′: No foods of animal origin | ||||
Control: typical diet in the United States | Vegetarian (col. 5): Exclude meat and fish | ||||
Omnivore: typical Western diet |
* Modified from Specker BL, Wey HE, Miller D: Differences in fatty acid composition of human milk in vegetarian and nonvegetarian women: long-term effect of diet, J Pediatr Gastroenterol Nutr 6:764, 1987. New England donors: vegetarians, 3 to 13 months postpartum; control subjects, 1 to 5 months; capillary gas-liquid chromatography columns.
† Modified from Sanders TA, Reddy S: The influence of a vegetarian diet on the fatty acid composition of human milk and the essential fatty acid status of the infant, J Pediatr 120:S71, 1992. British donors: 6 weeks postpartum; packed GLC columns.
The milk of strict vegetarians has extremely high levels of linoleic acid, four times that of cow milk (see Table 4-13 ). Some researchers include other long-chain fatty acids (e.g., C20:2, C20:3, C24:4, C22:3) as essential nutrients because they are structural lipids in the brain and nervous tissue. The effects of diet are also discussed in Chapter 9 .
One important outcome of linoleic and linolenic acids is the conversion of these compounds into longer-chain polyunsaturates. These metabolites have been shown to be important for fluidity of membrane lipids and prostaglandin synthesis. They are present in the brain and retinal cells. Long-chain polyunsaturates are needed for development of the infant brain and nervous system. When Gibson and Kneebore studied fatty acid composition of colostrum and mature milk at 3 to 5 days and later at 6 weeks postpartum, they reported that mature milk had a higher percentage of saturated fatty acids, including medium-chain acids, lower monounsaturated fatty acids, and higher linoleic and linolenic acids and their long-chain polyunsaturated derivatives.
Infant intake of fatty acids from human milk over the first year of lactation (solids were started at 4 to 6 months) was studied by Mitoulas et al. among mothers and infants in Australia. They determined the volume, fat content, and fatty acid composition of milk from each breast at each feed over 24 hours at 1, 2, 4, 6, 9, and 12 months. Volume of production was greater in the right breast (414 to 449 versus 336 to 360). Fat content also varied between breasts. Amounts of fat per 24 hours did not differ in the first year and only arachidonic acid and DHA differed between mothers. Changes in proportions of individual fatty acids may not result in commensurate changes in 24-hour infant intakes. The authors , note their findings were similar to Jensen’s work in 1995 in the United States.
When this same group of investigators in Australia (Kent et al. and Mitoulas et al. ) measured volume of milk, frequency of feedings, and fat content at 1 to 6 months of age in a normal group of mothers using demand feeding, they concluded infants should be fed on demand, day and night. They observed no relationship between total number of feeds and total volume. Furthermore fat content was 41.1 ± 7.8 g/L (range 22.3 to 61.6 g/L). Total fat was independent of frequency of feeding.
Prolonged lactation has long been suspected of providing reduced nutrition. It has been established that infection protection continues, but now there is evidence that high nutrition persists as well; 34 mother-baby dyads and 27 control dyads were studied by Mandel et al. The mothers who were breastfeeding beyond 1 year (12 to 39 months) were older (34.4 ± 5.1, years), lighter (59.8 ÷ 8.7 kg), and with lower BMI (22.1 ± 3.0) than controls (breastfeeding 2 to 6 months; age 30.7 ± 2.9 years, weight 66.3 ± 11.8 kg, and BMI 24.5 ± 3.9). Feeding frequency per day was 5.9 ± 3.3 versus 7.36 ± 2.65 (controls).The milk of mothers who were breastfeeding beyond a year had significantly increased fat and increased energy content compared with controls.
Factors affecting fatty acid composition include the stage of lactation, especially in specific fatty acids probably due to the recruiting of body fat stores. Milk from mothers of premature infants differs from that of mothers of full-term infants in fat content with higher levels of medium-chain fatty acids in premature milk. The significance of circadian rhythm on fatty acid composition is contradictory in the literature; therefore, studies of fat content should consider this in sample collection. Diet, on the other hand, has an extensive impact on fat content of milk with up to 85% derived from diet in the form of chylomicrons. This has led to dietary supplementation, especially utilizing the omega-3 fatty acids.
Brain Development
To address the issue of nutrition during brain development, it is important to consider the different periods of brain development that have been described biochemically. First, cell division occurs, with the formation of neurons and glial cells, and second, myelination. In the rat brain, 50% of polyenoic acids of the gray matter lipids were laid down by the fifteenth day of life. The fatty acids characteristic of myelin lipids appeared later. Gray matter is largely composed of unmyelinated neurons, whereas white matter contains a very high proportion of myelinated conducting nerve fibers. Normal brain function depends on both. The synthesis and composition of myelin can be influenced by diet in the developing rat brain.
Myelin-specific messenger ribonucleic acid (mRNA) levels are developmentally regulated and influenced by dietary fat. The neonatal response to dietary fat is tissue specific at the mRNA level.
The fatty acids characteristic of gray matter (C20:4 and C22:6) accumulate before the appearance of fatty acids characteristic of myelin (C20:1 and C24:1) in the developing brain. Arachidonic acid (C20:4) and DHA (C22:6) are synthesized from linoleic and linolenic acids, respectively, but the latter two must be obtained in the diet.
During the first year of life, the human brain more than doubles in size, increasing from 350 to 1100 g in weight. Of this growth, 85% is cerebrum; 50% to 60% of this solid matter is lipid. Cortical total phospholipid fatty acid composition in both term and preterm infants is greatly influenced by dietary fat intake. Phospholipids make up about one quarter of the solid matter and are integral to the vascular system on which the brain depends. Brain growth is associated with an increase in the incorporation of long-chain PUFAs into the phospholipid in the cerebral cortex. The transition from colostrum to mature milk leads to an increase in sphingomyelin and a decrease in phosphatidylcholine in the milk of mothers who deliver prematurely, along with a decrease in phospholipid content. Phospholipids are essential to brain growth, especially in a premature infant. Sphingomyelin and phosphatidylcholine are a source of choline, a major constituent of membranes in the brain and nervous tissue. Extreme dietary alterations in animal experiments have demonstrated an altered PUFA composition of the developing brain.
Such studies cannot be done in humans. Farquharson et al. therefore examined the necropsy specimens of cerebrocortical gray matter obtained from 20 term and 2 premature infants, all of whom died within 43 weeks of birth. All were victims of sudden death and were genetically normal. The infants had either received exclusively breast milk or exclusively formula. The latter group was divided by formula type into three groups: mixture of formulas, SMA, or CGOST (cow milk or Osterfeed). (SMA and CGOST are formulas or mixtures of formulas sold in the United Kingdom.) Breastfed infants had greater concentrations of DHA in their cerebrocortical phospholipids than formula-fed infants in all groups. A compensatory increase in n -6 series fatty acids (arachidonic, docosatetraenoic, and docosapentaenoic) occurred in the SMA group. No significant differences were seen between saturated and monounsaturated fatty acids. The two premature infants had the lowest levels of DHA.
Cerebrocortical neuronal membrane glycerophospholipids are composed predominantly (95%) of phosphatidylcholine, phosphatidylethanolamine, and phosphatidylserine. After birth, neuronal membranes and retinal photoreceptor cells derive most of their phospholipid DHA from diet and liver synthesis and not from fat reserves. Neither the liver nor the retinal and neuronal cells can synthesize DHA without reserves or a dietary supply. α-Linolenic acid, an EFA, is the precursor. If the enzymes are not activated or are inactivated by an excess of n -6 fatty acids, synthesis does not take place. Human milk provides the DHA and arachidonic acid.
Dietary supplementation with fish oil in the latter part of pregnancy resulted in increased DHA status at birth when measured in the umbilical blood. When postpartum women were supplemented with DHA by capsule in a blind study, breast milk levels of DHA ranged from 0.2% to 1.7% of total fatty acids, increasing with dose. Arachidonic acid levels and antioxidant status of plasma arachidonic acid and levels were unaffected.
Although DHA is essential to retinal development, levels peak in the retina at 36 to 38 weeks’ gestation, suggesting that the most rapid rate of retinal accumulation occurs before term. This further suggests that the premature infant is especially vulnerable to dietary deficiencies of DHA.
Dietary omega-3 (ω-3) fatty acids may not be essential to life, reproduction, or growth, but they are important for normal biochemical and functional development. Long-chain ω-3 fatty acids, DHA in particular, form a major structural component of biologic membranes. When the ratio of omega-6 (ω-6) is high compared to ω-3, fatty acids aggravate the deficiency. Studies in monkeys have shown that DHA deficiency affects water intake and urine excretion, as well as ω-3 fatty acid levels in red blood cells. Much remains to be learned about the effects of ω-3 fatty acids and DHA deficiency on developing human infants.
The EFAs, linoleic and linolenic acids, may have greater significance in the quality of the myelin laid down. Dick, observing the geographic distribution of multiple sclerosis worldwide, noted that the disease is rare in countries where breastfeeding is common. He postulated that the development of myelin in infancy is critical to preventing degradation later. Dick investigated the difference between human milk and cow milk in relation to myelin production in multiple sclerosis.
Experimental allergic encephalitis is a demyelinating condition and can be produced by shocking animals that have been sensitized to central nervous system (CNS) antigens. Newborn rats deficient in EFAs are more susceptible to this disease, which has been described as resembling multiple sclerosis pathologically.
Other Influences on Fat Content
Infections will alter milk composition. Mastitis does not alter fat content but does lower volume and lactose and increase sodium and chloride.
Parity has been cited as a major influence on fat content, with primiparous women having more fat than multiparous women. Prentice et al. found a significant relationship between fat content and triceps skinfold thickness. The authors found seasonal changes in The Gambia, where volume and fat were lowest following the rainy season, when nutrient resources are scarce.
Hyperlipoproteinemia
Milk from women with type I hyperlipoproteinemia has been investigated. Because the primary deficiency is serum-stimulated lipoprotein lipase in the plasma, resulting in reduced transfer of dietary long-chain fatty acids from blood to milk, levels of fat as fatty acids were abnormally low (1.5%) and the amounts of 10:0 and 14:0 higher than normal (see Chapter 16 ).
Cholesterol
Cholesterol is an essential component of all membranes and is required for growth, replication, and maintenance. Infants fed human milk have higher plasma cholesterol levels than formula-fed infants. Animal studies suggest that early postnatal ingestion of a diet high in cholesterol protects against high-cholesterol challenges later.
The cholesterol content of milk is remarkably stable at 240 mg/100 g of fat when calculated by volume of fat. The range, depending on sampling techniques, is 9 to 41 mg/dL. The amount of cholesterol changed slightly over time, decreasing 1.7-fold over the first 36 days, as reported by Harzer et al., and stabilizing at approximately day 15 postpartum at 20 mg/dL. This resulted in a change in the cholesterol/triglyceride ratio. The authors found no uniform pattern of circadian variations between mothers.
Neonatal plasma cholesterol levels range between 50 and 100 mg/dL at birth, with equal distribution of low-density lipoprotein (LDL) and high-density lipoprotein (HDL). Plasma cholesterol increases rapidly over the first few days of life, with LDL predominating regardless of mode of feeding. In breastfed infants, however, plasma cholesterol progressively increases compared with that in infants fed low to no cholesterol and high-PUFA formulas. This may have a lasting effect on the individual’s ability to metabolize cholesterol, a point yet to be confirmed. Low-birth-weight (LBW) premature infants are at risk for stimulation of endogenous cholesterol biosynthesis, resulting in marked elevations in plasma cholesterol as a result of intravenous nutrition.
The effect of breastfeeding on plasma cholesterol, body weight, and body length was studied longitudinally in 512 infants by Jooste et al. Breastfed infants had higher plasma cholesterol than the formula-fed infants, created by a direct mechanism that persisted for as long as the infants were breastfed. Body length was similar in breastfed and formula-fed infants, but formula-fed infants weighed more.
Cholesterol has been a factor of great concern because of the apparent association with risk factors for atherosclerosis and coronary heart disease. At present, commercial formulas have high P/S ratios and little or no cholesterol compared with those of human milk. Dietary manipulation does not change the cholesterol level in the breast milk. When the dietary cholesterol level is controlled, however, a fall in the infant’s plasma cholesterol level is associated with an increase in the amount of linoleic acid present in the milk.
Kallio et al. followed 193 infants from birth, measuring concentrations of cholesterol, very-low-density lipoprotein (VLDL), LDL, HDL2, and, on a limited group of 36 infants, HDL3 and apoprotein B. The largest differences between exclusively breastfed and weaned infants were at 2 months (0.8 mmol/L), 4 months (0.6 mmol/L), and 6 months (0.5 mmol/L). The LDL and apoprotein B concentrations were lower in weaned infants. VLDL and HDL3 were independent of diet. The authors concluded that the low intake of cholesterol and high intake of unsaturated fatty acids greatly modify the blood lipid pattern in the first year of life.
In a retrospective epidemiologic study of 5718 men in England born in the 1920s, 474 died of ischemic heart disease. The infant-feeding groups were divided into those breastfed but weaned before 1 year, breastfed more than a year, and bottle fed. The first group had the lowest death rate from ischemic heart disease and had lower total cholesterol, LDL cholesterol, and apolipoprotein B than those who were weaned after a year and especially those who were bottle fed. In all feeding groups, serum apolipoprotein B concentrations were lower in men with higher birth weights and weights at 1 year.
No long-range effect of serum cholesterol level has been identified, although Osborn described the pathologic changes in 1500 young people (newborns to age 20). He observed the spectrum of pathologic changes from mucopolysaccharide accumulations to fully developed atherosclerotic plaques. Lesions were more frequent and severe in children who had been bottle fed. Lesions were uncommon or mild in the breastfed children.
Animal investigations indicated that rats given high levels of cholesterol early in life were better able to cope with cholesterol in later life and maintained a lower cholesterol level.
In a study of six breastfed and 12 formula-fed infants, ages 4 to 5 months, Wong et al. measured the fractional synthesis rate. The breastfed infants had higher cholesterol intakes (18.4 ± 4.0 mg/kg/day) than formula-fed infants (only 3.4 ± 1.8 mg/kg/day). Plasma cholesterol levels were 183 ± 47 versus 112 ± 22 mg/dL; LDL cholesterol levels were 83 ± 26 versus 48 ± 16 mg/dL. An inverse relationship existed between the fractional synthesis rate of cholesterol and dietary intake of cholesterol. The authors concluded that the greater cholesterol intake of breastfed infants is associated with elevated plasma LDL cholesterol concentrations. In addition, cholesterol synthesis in human infants may be efficiently regulated by coenzyme A (CoA) reductase when infants are challenged with dietary cholesterol.
A carefully designed, well-controlled longitudinal study is needed to determine the long-range impact of cholesterol because it is a consistent constituent of human milk throughout lactation. The brain contains cholesterol especially in early development.
n -3 Fatty Acids
The n -3 fatty acids are important components of animal and plant cell membranes and are selectively distributed among the lipid classes. The role of DHA (22: n -3) in infantile nerve and brain tissue and retinal development has been discussed. It is also found in high levels in testis and sperm. Human milk contains DHA, and studies to evaluate the effects of “fish oil” supplements to the diet suggest an elevation of the dose-dependent levels.
Eicosapentaenoic acid (20: 5 n -3) is part of another group of n -3 fatty acids, the eicosanoids, which comprise two families: the prostanoids (prostaglandins, prostacyclins, and thromboxanes) and the leukotrienes. The prostanoids are mediators of inflammatory processes. Leukotrienes are key mediators of inflammation and delayed hypersensitivity. The eicosanoids are highly active lipid mediators in both physiologic and pathologic processes. Eicosanoids provide cytoprotection and vasoactivity in the modulation of inflammatory and proliferative reactions. Their precursors, long-chain PUFAs, can affect the generation of eicosanoids. The role of eicosanoids in physiologic and pathophysiologic processes is beginning to be identified. It clearly goes beyond adding a little DHA to the brew. Sellmayer and Koletzko reviewed this work.
In other species, restriction of n -3 fatty acids results in abnormal electroretinograms, impaired visual activity, and decreased learning ability. The influence of dietary n -3 fatty acids on visual activity development in very-low-birth-weight (VLBW) infants was evaluated by Birch et al., using visual-evoked response and forced-choice preferential-looking procedures at 36 and 57 weeks postconception. Feeding groups were randomized to one of three diets: corn oil (only linoleic), soy oil (linoleic and linolenic), and soy/marine oil (added n -3 fatty acids). The marine oil group matched the “gold standards” of VLBW infants fed human milk. Visual activity parameters in the other infants who did not receive n -3 oils were considerably lower.
The n -3 fatty acids appear to function in the membranes of photoreceptor cells and synapses. Jensen and Jensen suggest a daily intake of 18: 3 n -3 (0.5% of calories) with the inclusion of n -3 long-chain PUFA, which is available in human milk. Many studies affirm the value of n -3 fatty acids in the diet and as protection against heart disease, chronic inflammatory disease, and possibly cancer. When synthetic DHA and arachidonic acid are added to infant formula, the measurements of visual acuity do not match those of human milk. The tolerance for these formulas is still undocumented and long-range outcomes unreported.
Carnitine
Carnitine is γ-trimethylamino-β-hydroxybutyrate and is essential for the catabolism of long-chain fatty acids. Only two conditions in life have been described when carnitine is indispensable: total parenteral nutrition lasting more than 3 weeks and early postnatal life. In older individuals it is synthesized in the liver and kidney from the essential amino acids lysine and methionine. Carnitine serves as an essential carrier of acyl groups across the mitochondrial membrane to sites of oxidation and, therefore, has a central role in the mitochondrial oxidation of fatty acids in humans.
Newborns undergo major metabolic changes during transition from fetal to extrauterine life, including the rapid development of the capacity to oxidize fatty acids and ketone bodies as fuel alternatives to glucose. The fatty acids derived from high-fat milk and endogenous fat stores become the preferred fuel of the heart, brain, and tissues with high-energy demands. In addition, a dramatic increase occurs in serum fatty acids in the first hours of life. After the interruption of the fetoplacental circulation and in the absence of an exogenous supply of carnitine, neonatal plasma levels of free carnitines and acylcarnitines decrease very rapidly. Carnitine administration seems to act by increasing ketogenesis and lipolysis. When serum carnitine and ketone body concentrations were measured in breastfed and formula-fed newborn infants, lower carnitine levels were found in infants fed formulas than in those fed breast milk.
The levels of carnitine range from 70 to 95 nmol/mL in breast milk (up to 115 nmol/mL in colostrum) and from 40 to 80 nmol/mL in commercial formula (Enfamil). The bioavailability of carnitine in human milk may be a significant factor in the higher carnitine and ketone body concentrations in breastfed babies. In omnivorous mothers, carnitine levels do not vary considerably over time. Levels in the milk of lacto-ovovegetarian mothers were always consistently lower than those of omnivores. The lower serum level of lysine in these women is a possible cause of lower carnitine.
The carnitine levels in human milk were followed for 50 days postpartum and the mean level was found to be 62.9 nmol/mL (56.0 to 69.8 nmol/mL range) during the first 21 days and 35.2 ± 1.26 nmol/mL until days 40 to 50. Levels were not related to volume of milk secreted.
Proteins
All varieties of milks have been evaluated for their protein contents, which vary from species to species. Proteins constitute 0.9% of the contents in human milk and range up to 20% in some rabbit species. Proteins of milk include casein, serum albumin, α-lactalbumin, β-lactoglobulins, immunoglobulins, and other glycoproteins. Eight of 20 amino acids present in milk are essential and are derived from plasma. The mammary alveolar epithelium synthesizes some nonessential amino acids. Human milk amino acids occur in proteins and peptides, as well as a small percentage in the form of free amino acids and glucosamine ( Table 4-14 ; Figure 4-11 ).
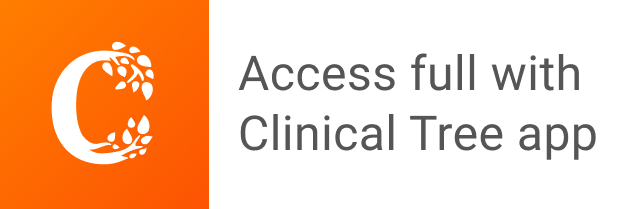