Structure and Function of the Normal Cell
All cells possess certain basic structural features, regardless of their location, type and function ( Fig. 9.1 ). The major division is into nucleus and cytoplasm.

Nucleus
The nucleus contains the chromosomes (see Chapter 1 ) and is surrounded by a nuclear envelope consisting of an inner and outer membrane, both of which are formed from a lipid bilayer. The nuclear envelope has pores which are used for the transport of RNA from the nucleus into the cytoplasm and for the import of nuclear proteins (which are made in the cytoplasm). Within the nucleus, one or more nucleoli are present. These sites mark the site of transcription of ribosomal RNA, which is a key component of ribosomes and is required in large amounts. In addition, ribosomal proteins are imported into nucleoli where they are assembled with ribosomal RNA to form ribosomes, which are then exported to the cytoplasm. The splicing machinery, or spliceosomes, are also located within the nucleus (see below). The messenger RNA (mRNA) is transported from the nucleus to the cytoplasm for translation. Nuclear RNA is a precursor of cytoplasmic ribosomal RNA (see Chapter 1 ).
Cytoplasm
The remainder of the cell is comprised by the cytoplasm. It is enclosed within a single cell or plasma membrane that is also a lipid bilayer and when stained has a distinctive trilaminar structure. The cell membrane has a very complex biochemical structure, including many proteins and lipids; it is not rigid, but can alter its shape in response to various stimuli. The major function of the cell membrane is control and maintenance of the appropriate intracellular electrolyte and biochemical environment by energy-requiring active transport mechanisms (e.g. sodium removal by the sodium pump). It also provides adhesion between adjacent cells and displays the individual cell’s major histocompatibility (transplant or human leukocyte antigen (HLA)) antigens. In some cells (e.g. polymorphs), it determines motility and phagocytosis.
The cytoplasm contains many organelles:
- 1.
Mitochondria are elongated, enzyme-rich bodies; each has a continuous external limiting membrane and an inner membrane folded into septa (cristae), which create partial subdivisions of the matrix. Oxidative phosphorylation occurs within mitochondria, which are able to oxidise proteins, carbohydrates and fats into energy, store it as adenosine triphosphate (ATP) and subsequently release it when required by the cell.
- 2.
Ribosomes are small ribonucleic acid protein particles (RNPs) that contain ribosomal RNA and ribosomal proteins. The ribosomal RNA and proteins are encoded by a large family of different genes within the nucleus. Ribosomes catalyse the synthesis of all proteins including those for intracellular metabolism. Aggregates of ribosomes are designated polysomes or polyribosomes.
- 3.
Spliceosomes are also RNPs that contain small nuclear RNAs and many proteins. They act upon pre-messenger RNA (pre-mRNA) to generate mRNA which is transported to the cytoplasm. Pre-mRNA is larger than mRNA and 90% of gene transcripts contain introns, which are removed by spliceosomes. Spliceosomes can also generate alternative mRNA transcripts from pre-mRNA which allows a single gene to encode multiple protein isomers.
- 4.
The endoplasmic reticulum (ER) is a complex network of intercommunicating narrow tubules and vesicles (cisternae) and is mainly responsible for N-linked glycosylation and the transport of certain proteins to the Golgi apparatus. Two continuous types of ER exist: rough ER, where ribosomes are attached to the outer surface, and smooth ER where ribosomes are absent. The rough ER is continuous with the outer membrane of the nucleus. The smooth ER is involved in the synthesis of lipids, including cholesterol and phospholipids. In certain cell types, the smooth ER plays an important role in the synthesis of steroid hormones from cholesterol.
- 5.
The signal recognition particle (SRP) is another ribonucleic acid particle and is responsible for identifying those proteins that need to be secreted into the lumen of the ER for subsequent transport to the Golgi apparatus and to different parts of the cell. Proteins that enter the ER lumen usually contain a signal recognition peptide at their N-terminal that is recognised by the SRP, which directs the protein/ribosome complex to the ER for translocation ( Fig. 9.2 ).
Fig. 9.2 Sequence of events in protein synthesis. - 6.
The Golgi complex is a stack of flattened membranous sacs and is the major sorting centre of the cell. Proteins are transported from the Golgi complex to lysosomes, secretory granules or the cell membrane according to signals within their amino acid sequences and how they associate with other proteins and lipids. The Golgi apparatus also adds O-linked sugars to proteins and may modify the N-linked sugars that arrive from the ER.
- 7.
The centrosome is a relatively clear area, usually near the cell centre, containing two centrioles. Centrioles are hollow cylindrical bodies, 0.3 to 0.7 μm in length, which replicate before mitosis and orientate the mitotic spindle.
- 8.
Lysosomes are round or oval membrane-bound bodies containing proteolytic enzymes (acid hydrolases) for digesting unwanted endogenous and phagocytosed exogenous material.
- 9.
Phagosomes are membrane-bound bodies containing material ingested by phagocytosis. To effect digestion, phagosomes combine with lysosomes to produce phagolysosomes. When indigestible material remains, residual or dense bodies are formed.
- 10.
Filaments . Cells have microfilaments (made of actin and 7 nm in diameter), intermediate filaments (made of one or more of the family of over 50 intermediate proteins, 8 to 10 nM in diameter) and microtubules (made of tubulin, 25 nM in diameter). Filaments are of indefinite length and have a variety of functions. For example, tonofibrils are a type of intermediate filament that converge on intercellular junctions (desmosomes) to promote cell adhesion; actin microfilaments interact with myosin to generate force in muscles cells or interact with myosin motors in other cell types to effect cell movement or vesicle transport; microtubules radiate from centrioles and are important for mitosis and vesicle transport.
- 11.
Clathrin coated pits . Cells use a variety of mechanisms to endocytose cell surface proteins and lipids and the use of clathrin coated pits is one of the best characterised processes. Some cell surface proteins, such as transferrin, are selectively endocytosed in a network of clathrin, known as a clathrin pit. The endocytosis of transferrin allows the entry of iron into a cell. Low-density lipoproteins are also endocytosed via clathrin pits.
- 12.
Vesicles are small spheres containing specialised cytoplasm that is enclosed by a lipid bilayer. They are used for transport and communication between different parts of the cells. For example secretory vesicles (exosomes) are used to transport proteins from the ER to the Golgi and to secrete proteins. Vesicles known as endosomes are essential for endocytosis and transport proteins from outside the cell to the cytoplasm or to lysosomes.
- 13.
Proteasomes are large protease complexes within the cytoplasm that degrade proteins into amino acids, which are recycled. Protein degradation is regulated by the attachment of a small highly conserved protein called ubiquitin which directs the tagged protein to the proteasome.
Specific structures are unique to, and characteristic of, specialised cells (e.g. myofilaments in muscle cells and melanosomes in melanocytes). Several other structures may also be seen, including glycogen granules, lipofuscin granules, myelinoid bodies, siderosomes and lipid droplets.
Cell Types
The human body contains over 200 mature cell types that are sometimes classified into four tissue types: epithelial, connective, muscle or nervous. Alternative classifications place more emphasis upon the origin of a mature cell type from the three layers of the early embryo, namely ectoderm, endoderm or mesoderm. Tissue membranes are composed of either epithelial or connective cells.
Epithelial cells cover or line body surfaces and internal cavities; in addition, most glands are epithelial, being derived embryologically from body surfaces. Epithelial cells therefore act as selective and protective barriers and synthesise most secretions.
Connective tissue cells are derived largely from the embryonic mesoderm. Connective tissue exists in many types, and its composition varies in different parts of the body, depending on local requirements. Its main function is to provide structural support, generally as fibrous tissue and specifically as bone, cartilage, muscle and tendon. It is probably also responsible for body defences since leucocytes and mononuclear phagocyte (reticuloendothelial) system cells are usually considered connective tissue in origin.
Intercellular Matrix
The intercellular matrix refers to the non-living material filling the space between cells. This varies considerably in amount; very little is seen between epithelial cells, whereas connective tissue cells are often quite widely separated by matrix, the exact nature of which may provide the unique connective tissue structure (e.g. bone and cartilage). Interstitial extracellular fluid is located in the intercellular matrix.
The epithelial intercellular matrix is a narrow, mucopolysaccharide-rich layer traversed by intercellular junctions. Formerly a designated cement substance, it is now thought, in some instances, to be an integral component of the cell membrane’s external surface.
Connective tissue intercellular matrix contains ground substance and fibres. The ground substance is a gel of variable consistency and viscosity, containing mucoproteins, glycoproteins and mucopolysaccharides; it is probably mainly secreted by fibroblasts. Collagen is one of the most important fibres and provides structural rigidity. It has a tri-helical structure derived from a soluble precursor (procollagen), secreted by fibroblasts and osteoblasts via an insoluble intermediate (tropocollagen). Four collagen types, encoded by different structural genes, are known: collagen type I is the form found in bone, collagen type II is found in cartilage and collagen type IV is found in the basement membranes of epithelia. Collagen type III is found in the tissues of the fetus but this is replaced by type I following birth. Adults have little type III, although it does reappear during the wound response. Collagen type I is a major component of bones, skin and a number of other tissues and this single protein comprises more than 50% of the total protein in the body.
Proteins, Peptides and Amino Acids
Each of the many cell types in the body makes a unique set of proteins. There is considerable variation in the types of protein made by each cell type and a particular cell synthesises is only a part of the total human protein repertoire. For example, despite the large amount of albumin present in blood plasma, it is only synthesised in adults by hepatocytes in the liver. This is despite the fact that every cell contains within its nucleus a copy of the gene for albumin along with a copy of every other human gene. During development and differentiation, the DNA within each cell type comes under a regulatory mechanism such that some genes are expressed and others are repressed. This mechanism underlies the concept of ‘totipotency’, which refers to the ability of totipotent stem cells to generate all of the many different mature cell types during differentiation. In the case of some proteins, expression does not occur all the time but does so in response to a specific signal such as a hormone. The control of protein expression is aberrant in many tumours and inappropriate proteins are produced.
The proteins synthesised by a cell play a number of different roles. Some proteins have a structural role. For example, there are proteins that provide the structural basis for the membrane around the cell and the membranes around the nucleus, mitochondria and the other discrete subcellular organelles (see Fig. 9.1 ).
Other proteins such as enzymes have non-structural roles. The human genome encodes many thousands of proteins which act as enzymes for specific reactions; these include synthesis reactions, degradative reactions, energy-producing reactions and energy-storing reactions. Very few biochemical reactions occur in the absence of enzymes and thus this catalysis is essential for life.
Other non-structural proteins include hormones, neurotransmitters and transport proteins, all of which are found in plasma. One of the functions of albumin is to transport free fatty acids, and the plasma protein transferrin carries iron from the gut to tissues. In blood, there are different classes of lipoprotein that carry lipids in circulation; chylomicrons carry triglycerides from the gut to adipose tissue and the liver. Low-density lipoproteins carry much of the cholesterol that is required by tissues; some of the cholesterol comes from dietary sources but most has been synthesised in the liver.
Some proteins in plasma play a hormone-binding role. Other major constituents of plasma are the immunoglobulins (antibodies) and complement proteins that are part of the immune system.
Amino Acids
There are 21 amino acids used in the synthesis of proteins. The generalised structure of an amino acid is shown in Fig. 9.3 ; each amino acid has a characteristic R group or side chain. Fig. 9.4 shows the chemical structure of each amino acid used by humans, along with its three-letter and one-letter designation. Every amino acid has a mirror image form but only L amino acids (not the D amino acids) are constituents of proteins. Similarly, monosaccharides such as glucose have mirror image forms but conversely the six carbon sugars used in nature are D-isomers not L-isomers.




Selenocysteine is a recently discovered and unusual 21st amino acid. It has the same structure as cysteine except that it has selenium instead of a sulphur atom (Fig 9.3). Unlike the other 20 amino acids, there is not a dedicated codon (or codons) that encodes selenocysteine. However, for the small number of human proteins that contain selenocysteine, this amino acid is encoded by a UGA stop codon (see Chapter 1) but only when a selenocysteine insertion sequence is also present in the mRNA. Not all amino acids can be synthesised in vivo . Those that can be synthesised are referred to as the ‘non-essential amino acids’ and comprise the following:
- •
Alanine
- •
Aspartic acid
- •
Asparagine
- •
Glutamine
- •
Cysteine
- •
Glutamic acid
- •
Glycine
- •
Proline
- •
Serine
- •
Tyrosine.
The essential amino acids are:
- •
Arginine
- •
Histidine
- •
Isoleucine
- •
Leucine
- •
Lysine
- •
Valine
- •
Methionine
- •
Phenylalanine
- •
Selenocysteine
- •
Threonine
- •
Tryptophan.
The situation is slightly more complex than this since cysteine can be synthesised if there is sufficient methionine present; similarly, tyrosine can be synthesised if there is sufficient phenylalanine present. Histidine and arginine are not strictly essential but are required for normal growth. The essential amino acids are required in the diet. A simple estimate of total protein will not indicate sufficiency. For example, if the diet contained insufficient valine, then the total protein content would be immaterial since protein synthesis cannot continue in the absence of valine. This leads to the concept of qualitative and quantitative dietary sufficiency. A diet is only satisfactory if it contains adequate concentrations of all the essential amino acids. A protein is a sequence of amino acids that are chemically coupled by the enzyme activity of ribosomes. The sequence of events that occurs in the synthesis of a protein is depicted in Fig. 9.2 . The sequence of amino acids for a protein is encoded in the gene for that protein. This genetic information is stored in the form of DNA. The structure of DNA is that of a double helix of two long nucleotide chains. Each chain has a deoxyribose phosphodiester backbone carrying a covalently linked sequence of the nucleotides or bases that make up the genetic code. There are four bases, namely, adenine, cytosine, guanine and thymine. The strands of DNA are held together tightly by hydrogen bonding between bases on each strand. The base adenine bonds to thymine and cytosine bonds to guanine. This complementarity provides accurate and strong pairing and is the basis of the famous discovery by Watson and Crick of the structure of DNA.
There are many hundreds of genes on each chromosome and they are found on both DNA strands. A gene has a specific sequence and number location within each chromosome. The first stage in the production of a protein is transcription; this is the term used to denote the process by which a complementary copy of the gene is made. The initial transcription product is called pre-mRNA, which is rapidly processed to mRNA by splicing. mRNA is a single-stranded nucleic acid that has ribose rather than deoxyribose in the phosphodiester backbone. Like DNA it contains the bases cytosine, guanine and adenine but, in contrast to DNA, contains uracil rather than thymine. The terminology of the bases is complex. The terms ‘adenine’, ‘cytosine’, ‘guanine’, ‘thymine’ and ‘uracil’ are used to describe the bases. When these are linked to the sugar ribose they are called ‘adenosine’, ‘cytidine’, ‘guanosine’, ‘uridine’ and ‘thymidine’. If they are linked to deoxyribose, the prefix ‘deoxy’ is used.
When mRNA is transported out of the nucleus, it becomes attached to ribosomes. Each functional ribosome is composed of two subunits (known as large and small or 50 S and 30 S), each of which contains a number of different proteins and RNA species. Together this complex is responsible for the recognition of all the substrates and factors that are required for protein synthesis. Ultimately, the function of the ribosome is to couple together successive pairs of amino acids through the formation of peptide bonds ( Fig. 9.5 ) in accordance with the genetic code. Ribosomal RNA is expressed by all animal and plant species and is highly conserved. The comparison of ribosomal RNA sequence between species is the basis of many taxonomy and phylogenetic studies.

Structure of Proteins
Proteins vary greatly in size. Small proteins are referred to as ‘peptides’ and some of these have fewer than 10 amino acids in their sequence and have molecular weights of about 1000 Da. Examples of peptides include the hormones oxytocin and vasopressin. At the other end of the spectrum, some proteins are close to 1 million Da in molecular weight and have hundreds of amino acids in their sequence. Examples of larger proteins would be α 2 -macroglobulin, an important anti-protease found in plasma, and the IgM class of immunoglobulins, which play a role in the early defence reaction against infecting organisms.
Proteins have primary, secondary, tertiary and often quaternary structures. The primary structure is the simplest description of the protein and refers to the linear sequence of amino acids, which is encoded by the gene for that protein. The primary sequence begins with an amino acid with a free amino terminal ( N -terminus) and concludes with the last amino acid, which will have a free carboxyl group ( C -terminus). All the intervening amino acids lose their free amino and carboxyl groups during the formation of the peptide bonds (see Fig. 9.5 ). Some proteins are modified at the N -terminus. For example, some of the proteins involved in the blood-clotting cascade have the N -terminal glutamic acid modified to become a γ-carboxyglutamic acid residue. The blocking of the N -terminus in this case involves an enzyme cascade that requires vitamin K. Warfarin acts by inhibiting this pathway. Proteins that enter the lumen of the ER often have signal peptides of about 20 amino acids that are recognised by the SRP and are then subsequently removed by signal peptidase (see Fig. 9.2 ).
The tertiary structure of a protein is the three-dimensional arrangement of a single protein and this structure is determined by the linear or primary amino acid sequence. There has been considerable progress in the prediction of the three-dimensional protein structure from the linear gene sequence but experimental verification through techniques such as x-ray crystallography are still essential. Nuclear magnetic resonance can also be used to determine the structure of smaller proteins. The tertiary structure of a single protein can be subdivided into elements of secondary structures, namely alpha helices, beta sheets, turns and loops. Alpha helices and beta sheets are stabilised by regular hydrogen bonding between the repeating CO and NH groups of the peptide backbone. This leaves the amino acid side chains sticking out which allows them to interact with each other (by hydrogen bonding or ionic attraction) or to catalyse chemical reactions. The side chains of the amino acid cysteine can form covalent disulphide bonds; these are commonly found on proteins on the outside of a cell. The overall tertiary structure of a protein is determined by the interaction of its secondary structures and in addition the tertiary structure of a soluble protein is also stabilised by its hydrophobic core, which is formed by the interaction of amino acids with hydrophobic side chains. Loop regions are less structured and are nearly always found at the cell surface, these regions often contain amino acids that have key catalytic functions. Turns are so named because they stabilise a change in direction of a peptide chain.
Many proteins, such as haemoglobin, are composed of subunits. These can be identical or dissimilar. The subunits are held together by non-covalent forces which are most commonly charge–charge interactions. The three-dimensional structure of all the subunits comprising a protein is referred to as its quaternary structure. A new technique called cryo-electron microscopy can be used to determine the structure of higher-order protein complexes.
Purification and Analysis of Proteins
There are several techniques available for the separation of proteins. Most can be used for the preparative purification of a single protein to homogeneity as well as analytically in order to determine the degree of purity of a protein.
Some methods separate proteins on the basis of size. Thus, gel permeation chromatography involves the use of beds of resin beads that contain pores of a predetermined size. Some proteins will diffuse into these pores while other proteins are too large and are excluded. Large proteins are eluted from the bed before the smaller ones. A second method involving size is gel electrophoresis. A support of agarose or polyacrylamide is used and the protein solution is exposed to an electric field. In the absence of detergent, the proteins will move according to their mass/charge ratio. If a detergent such as sodium dodecyl sulphate is added to the system, the proteins move at a rate proportional to their size alone.
Ion-exchange chromatography involves the use of resins that contain at their surface positively or negatively charged groups. Proteins contain negatively charged carboxyl groups and positively charged amino groups. The proteins will bind to the resin and can then be eluted by changing either the pH or the salt strength of the buffers used. Individual proteins have unique patterns of charge and can be separated from one another if gradients of buffer strengths are used.
Another technique called affinity chromatography can often be used to achieve complete purity of a protein in a single step. Here a chemical moiety is coupled to a bed of support beads. The agent that is coupled binds with high specificity to the protein that is to be purified. When a mixture of proteins in solution is passed through the bed, only the target protein binds to the beads and, after washing off all non-specifically bound material, a high salt concentration or change of pH is used to elute the protein. The types of ligand that can be coupled to the beads are antibodies or substrate for an enzyme.
Modification of Protein Structure
Very few proteins are composed purely of amino acid chains and most have carbohydrate chains covalently attached. This is referred to as post-translational modification because, after the protein has been synthesised on the ribosomes, the peptide passes to the ER and then the Golgi apparatus, where enzymes assemble chains of sugars onto the protein.
The carbohydrate is always linked to specific amino acids in the protein chains, namely serine, threonine or asparagine. In the case of serine or threonine, the carbohydrate is linked via the oxygen of the hydroxyl groups and is hence referred to as O -linked carbohydrate. In the case of asparagine, it is linked to the nitrogen of the amino group and is referred to as N -linked carbohydrate. N-linked glycosylation occurs in both the ER and Golgi, whereas O-linked glycosylation occurs in the Golgi.
There is considerable diversity in terms of the size and nature of the carbohydrate that is attached to protein and it appears to serve different functions. Proteins that are part of membranes are heavily glycosylated (the term used to denote the attachment of sugar residues) and the oligosaccharide chains play a role in maintaining the proteins in the correct orientation within the membrane. Proteins in the cytosol are also often glycosylated.
Proteins found in plasma are also often highly glycosylated and in this case the sugar plays a regulatory role in controlling turnover. While sugar chains remain intact, the protein continues to circulate in plasma. When the sugar chains become cleaved or modified, then the proteins are removed from circulation and degraded. The liver has a most efficient mechanism for detecting altered circulating proteins. There are in the newly formed proteins no terminal galactose residues; there is a sialic acid residue after the galactose. If the galactosyl groups are revealed following damage to the protein, it is immediately removed from circulation by hepatocytes which contain at their surface a receptor for the terminal galactose.
Metabolism
Overall Energy Metabolism
Every cell has to maintain an adequate supply of energy. Dietary lipids, carbohydrates and proteins are all sources of energy because each has an intrinsic energy that is released when the molecule is broken into smaller parts. Nature has evolved a mechanism by which this energy can be harnessed to produce ATP, which is used to drive most biological reactions. Which particular energy source is used depends upon a number of parameters such as dietary status, circadian rhythm, etc. In this section, the individual metabolic pathways will be described as will the controls that operate and the interrelationships between the pathways.
The metabolism of carbohydrates (sugars), fats (fatty acids and glycerol) and protein begins with pathways that are specific for each energy source. The products from these pathways then feed into common pathways. The overall interaction of the pathways is shown in Fig. 9.6 .

Sugars, fatty acids and amino acids are all metabolised to produce acetate in the form of acetyl coenzyme A (acetyl-CoA). The acetyl group has to be covalently linked to CoA for stabilisation.
The acetyl-CoA then enters the tricarboxylic acid (TCA) cycle, which is also known as the ‘citric acid cycle’ or the ‘Krebs cycle’, after the biochemist who was involved in its discovery. The TCA cycle results in the complete degradation of acetyl groups. The products are carbon dioxide, which is breathed out by the lungs, and water. During this process hydrogen becomes available in the form of nicotinamide adenine dinucleotide (NADH) and flavin adenine dinucleotide (FADH 2 ). NADH and FADH 2 are fed into the respiratory chain (also known as the electron transport chain) inside the mitochondrion in order to produce ATP from adenosine diphosphate (ADP). This reaction within the respiratory chain requires molecular oxygen, hence the name ‘respiratory chain’.
The overall oxidation of glucose is:
That is, the complete oxidation of a molecule of glucose to carbon dioxide and water. Nature has evolved an efficient sequence of reactions and much of the energy within the glucose molecule is utilised in the production of ATP from ADP. A single molecule of glucose can result in the net formation of 2 ATP molecules by glycolysis and a further 28 molecules of ATP are generated by oxidative phosphorylation within the mitochondria. The chemical combustion of glucose in the presence of excess oxygen yields 686,000 cal/mol. Each time an ADP molecule is converted to one of ATP, 7300 cal are captured. Because there is a net profit of about 30 molecules of ATP, this indicates that the process is approximately 32% efficient. The remaining energy is released as heat.
Glycolysis
The enzymes of the glycolytic pathway are found in the cytoplasm of the cell. The glycolytic pathway converts a molecule of glucose that contains six carbon atoms to two molecules of pyruvic acid, each containing three carbon atoms. The pathway for glycolysis is shown in Fig. 9.7 and it can be seen that in the first few steps the glucose becomes doubly phosphorylated; this consumes ATP and is thus energy dependent. Glucose is converted to glucose 6-phosphate, which is in turn isomerised to fructose 6-phosphate. Here, isomerisation refers to the rotation of two bonds around a carbon atom. The fructose 6-phosphate is then phosphorylated to fructose 1,6-diphosphate and this is then hydrolysed to produce one molecule of 3-phosphoglyceraldehyde and one of dihydroxyacetone phosphate.

The next step is the conversion of the dihydroxyacetone phosphate into 3-phosphoglyceraldehyde. Thus, two molecules of 3-phosphoglyceraldehyde have been generated from one molecule of glucose. These two molecules are then converted to pyruvic acid via the intermediate stages 1,3-diphosphoglyceric acid, 3-phosphoglyceraldehyde and phosphoenolpyruvic acid. The two steps involving the metabolism of 1,3-diphosphoglyceric acid and phosphoenolpyruvic acid are worthy of note. In both cases, the phosphate group that is transferred is of a ‘high-energy’ type (this means that the phosphate bonding has high internal energy). These groups are transferred to ADP to generate ATP.
Although more ATP is formed during glycolysis than ATP expended, there is a net production of only two molecules of ATP. Unlike the respiratory chain which is where the bulk of cellular ATP is produced, the glycolytic pathway does not require oxygen. Thus, for short periods of time the cell can survive without consuming oxygen by generating ATP via glycolysis. In the absence of oxygen, pyruvate is converted to lactate in order to regenerate NAD + . The lactate generated by anaerobic glycolysis is often secreted by cells and can be used by the liver to make glucose by gluconeogenesis (Cori cycle). Alternatively lactate is used as a fuel for the TCA cycle by cells undergoing aerobic glycolysis.
Citric Acid Cycle
The enzymes that carry out the citric acid cycle are located inside the mitochondria. Pyruvate is transported into the mitochondrion and is oxidatively decarboxylated by the pyruvate dehydrogenase complex to form Acetyl CoA; this process also generates CO 2 and NADH
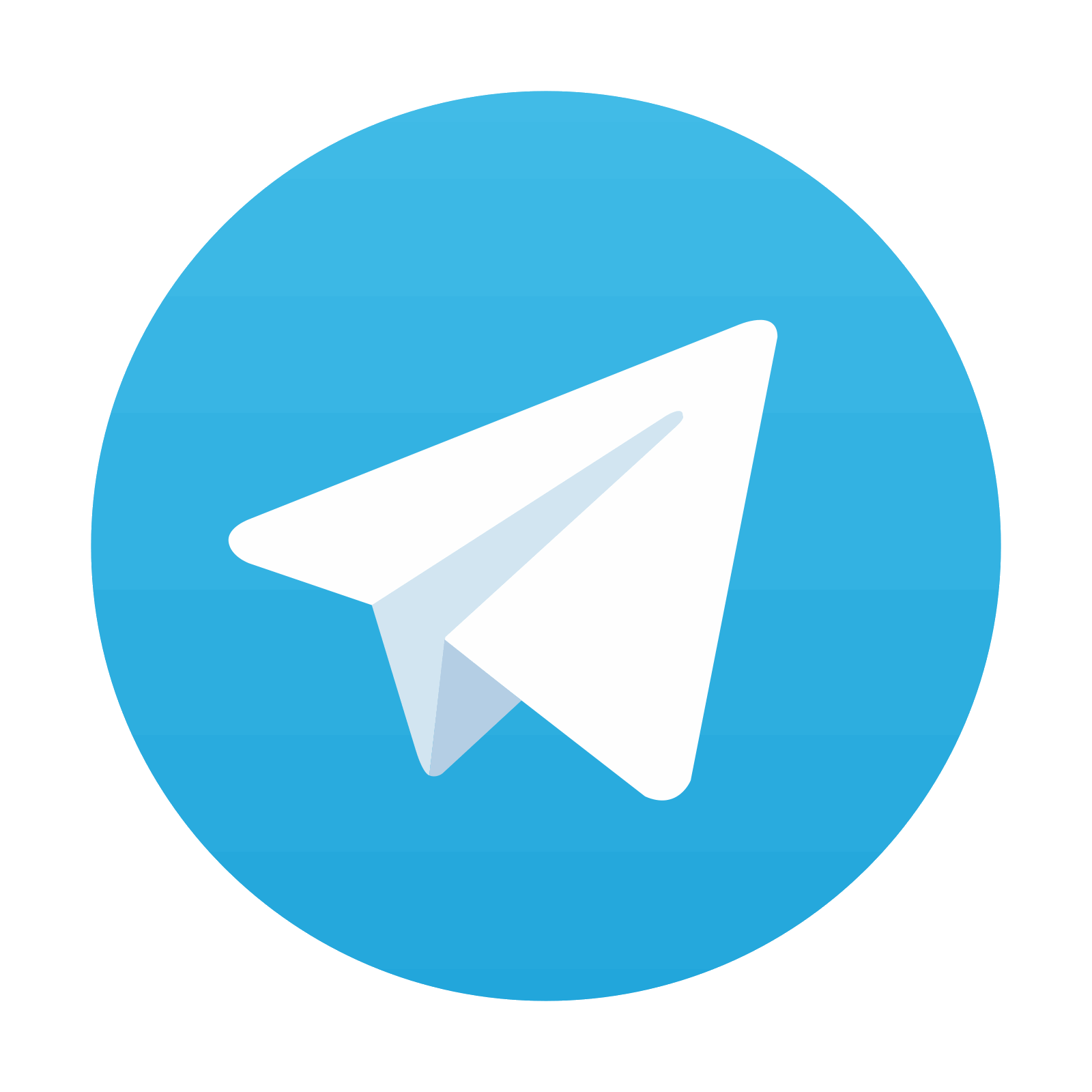
Stay updated, free articles. Join our Telegram channel
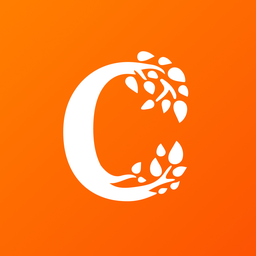
Full access? Get Clinical Tree
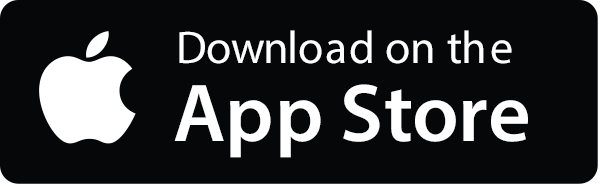
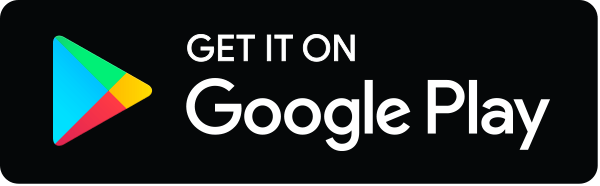