Anemias
Erythroid Development
Early hematopoietic cells originate in the yolk sac. By the eighth week of gestation, more definitive fetal erythropoiesis is taking place in the liver. The liver remains the primary site of erythroid production throughout the early fetal period. By 6 months of gestation, the bone marrow becomes the principal site of erythroid cell development. Later during gestation, a switch occurs in the type of hemoglobin being formed, with adult hemoglobin (HbA) re-placing fetal hemoglobin (HbF). The site of production of erythropoietin (EPO) switches from the less sensitive hepatic to the more sensitive renal site (1).
The earliest characterized erythroid precursor is the burst-forming unit (BFU-E), which gives rise to colony-forming units (CFU-E). These are identified by their growth characteristics in culture. Neonatal BFU-E and CFU-E are as sensitive as their adult counterparts to EPO stimulation (2,3). Earlier development is a function of other factors, including interleukin-3 and stem cell factor. The numbers of BFU-E progressively decline along the series of fetal blood, cord blood, adult bone marrow, and adult blood. More than 40 times the number of BFU-E can be cultured from fetal blood as from an equivalent volume of adult blood (1). Measurement of the peripheral blood pool does not indicate the size of the total body pool, so it cannot be inferred that the fetus has a markedly greater erythropoietic potential than an adult does (4). It is probably at least comparable.
The major difference between fetal and adult erythropoiesis is in the response to EPO. Erythropoiesis is controlled by a feedback loop involving EPO. A decrease in erythrocyte mass is reflected by an increase in EPO, which drives erythropoiesis to increase erythrocyte mass and diminish EPO production. The expected correlation between EPO and measures of oxygen delivery (e.g., hemoglobin level, mixed venous oxygen tension, and available oxygen) can be detected in premature neonates, providing evidence that the same feedback loop exists (5-,6 and 7). The measured levels of EPO are much lower than those of older children and adults with corresponding degrees of anemia. Brown and colleagues (7) presented evidence that the magnitude of the EPO response was lowest in the least mature infant (27 to 31 weeks of gestation). Forestier and associates (8) found low EPO values in cordocentesis samples from infants between 18 and 37 weeks of gestation. Surprisingly, there was no correlation between gestational age and EPO level. This poor EPO response persists through the neonatal period, resulting in a reduced erythropoietic stimulus and lower hemoglobin levels in premature infants.
Normal Hemoglobin Levels
In the newborn period, the hemoglobin concentration is undergoing constant physiologic change. A clear definition of the normal hemoglobin range is therefore important for proper evaluation and management.
Normal hemoglobin values at birth have been determined through measurement of levels in cord blood of newborn infants. In a review of normal blood values in the newborn, Oski and Naiman (9) cited a range of 13.7 to 20.1 g/dL, with a mean of 16.8 g/dL. Blanchette and Zipursky (10) obtained similar results in studies of healthy newborns, yielding cord hemoglobin values (mean ± 1 SD) of 16.9 ± 1.6 g/dL in full-term infants and 15.9 ± 2.4 g/dL in premature infants. Definitive values for premature infants are known as a result of cordocentesis sampling. Data from Forestier and colleagues (8) for 18 to 29 weeks of fetal life and our data for fetuses older than 36 weeks of gestation are given in Table 46-1. Based on these data, cord hemoglobin levels less than 13.0 g/dL should be considered abnormal in term and premature (<36 weeks of gestation) neonates. In the very premature infant (<26 weeks of gestation), values as low as 12.0 g/dL may be acceptable. If anemia is confirmed, a prompt and careful search for the cause should be initiated.
One factor that can significantly influence the hemoglobin level in newborn infants is the amount of placental transfusion. At birth, blood is rapidly transferred from the placenta to the infant, with one-fourth of the placental transfusion occurring within 15 seconds of birth and one-half by the end of the first minute (11). The placental vessels contain 75 to 125 mL of blood at birth.(12) Usher and associates (13) demonstrated that the blood volume of an infant could be increased by as much as 61% by delayed cord clamping. In that study, the average erythrocyte mass in a group of infants with delayed cord clamping was 49 mL/kg at 72 hours of age compared with 31 mL/kg in a group in whom the cord was clamped immediately after birth. Although infants delivered after delayed cord clamping have higher hemoglobin values, it should be recognized that the placental transfusion may be markedly reduced or prevented if the infant is held above the level of the placenta at the time of delivery; in this situation, it is possible for an infant to lose blood into the placenta and be born anemic (14).
TABLE 46-1 NORMAL ERYTHROCYTE VALUES DURING GESTATIONa | ||||||||||||||||||||||||||||||
---|---|---|---|---|---|---|---|---|---|---|---|---|---|---|---|---|---|---|---|---|---|---|---|---|---|---|---|---|---|---|
|
Anemia in the Newborn Period
Anemia at birth or appearing during the first few weeks of life can be broadly categorized into three major groups. The anemia may be the result of blood loss, hemolysis, or underproduction of erythrocytes.
Physiological Anemia and the Anemia of Prematurity
The hemoglobin concentration of healthy full-term and premature infants undergoes typical changes during the first weeks of life (15,16,17 and 18). After birth, there is a transient increase in hemoglobin concentration as plasma moves extravascularly to compensate for the placental transfusion and an increase in circulating erythrocyte volume that occurs at the time of delivery (19). Thereafter, the hemoglobin concentration gradually falls, to reach minimal levels of 11.4 ± 0.9 g/dL in term infants by 8 to 12 weeks of age and 7.0 to 10.0 g/dL in premature infants by 6 weeks of age (Fig. 46-1) (18).
There are several reasons for the fall in hemoglobin. The first is the decline in erythrocyte production that occurs in the first few days of life and is evidenced by a fall in reticulocyte counts during that time (Fig. 46-2). Normally, reticulocyte counts may be elevated during the first 1 or 2 days of life (200 to 300 × 109/L) but then fall to low levels (in the order of 50 × 109/L) through the remainder of the neonatal period. This diminution of erythropoiesis is probably related to decreased EPO production. The reduced EPO response persists until approximately 6 weeks of age, at which time erythrocyte production increases, as evidenced by a sharp rise in reticulocyte numbers in the blood and an increase in total body hemoglobin (Fig. 46-2). Other factors that contribute to the physiologic anemia in newborns, particularly the more profound anemia in premature infants, are the shortened survival of neonatal erythrocytes and rapid body growth (Fig. 46-2) (20,21).
The effect of rapid body growth on hemoglobin levels is unique to neonates and deserves special mention. Healthy premature infants are in a phase of rapid growth when active erythropoiesis, as evidenced by a mild reticulocytosis, resumes at 6 to 8 weeks of age. Associated with this rapid gain in body weight is an obligatory increase in the total circulating blood volume. The resultant hemodilution may cause a peripheral hemoglobin concentration that is static or even falls slightly. The apparent paradox of a stable or falling hemoglobin concentration despite active erythropoiesis (i.e., mild reticulocytosis and an increasing erythrocyte mass) gradually corrects, and the peripheral hemoglobin concentration increases (see Fig. 46-2). Failure to recognize the important effect of rapid body growth on the peripheral hemoglobin concentration may lead to inappropriate investigation and treatment of apparent anemia (16,22).
The signs and symptoms of this early anemia in premature infants are nonspecific and reflect changes in metabolic rate or cardiorespiratory function and perfusion. Controversy exists about whether tachycardia, tachypnea, periodic breathing, and apnea are reliable indicators of anemia (23,24,25,26,27,28 and 29). Stockman and Clark (30) demonstrated an improvement in weight gain after transfusion in premature infants in infants with poor weight gain. Others have not confirmed these observations (27,28 and 29). Lactate measurements and cardiac output can be shown to decrease following transfusion, but neither measurement on its own can be considered a surrogate for diagnosing anemia (27,31).
Trials of folate, iron, and vitamin E have not shown any evidence of benefit in preventing the physiological anemia of infancy (32). Optimal protein supplementation is important for maintaining good hematopoiesis (33,34,35,36,37 and 38).
Several groups have tested blood transfusion as therapy for the anemia of prematurity. Blank and associates (39) and Meyer and associates (40) were unable to demonstrate clinical benefit in transfused versus nontransfused neonates other than an improved hematocrit. In both studies several children were transfused for tachycardia, apnea, bradycardia, or other clinical signs interpreted as signs and symptoms of anemia. Others have shown benefit in these clinical parameters from transfusion in selected asymptomatic infants (41,42 and 43), although hemoglobin values are not necessarily the best indicator of need (41). Surveys of blood product use in neonatal units show that most transfusion practices are based on a preset hemoglobin value or what the clinician interprets as signs and symptoms of anemia (apnea, poor weight gain, etc.) (44). Similar clinical criteria have been incorporated into guidelines for the transfusion of premature infants (45). Trials of transfusion therapy at arbitrary values have not demonstrated clear benefit and may expose infants to infectious agents (e.g., cytomegalovirus [CMV], hepatitis, human immunodeficiency virus [HIV]) (46) and other risks from blood products (e.g., graft-versus-host disease). Future trials of transfusion therapy for anemia in premature infants require better defined end points before they can yield definitive results. Prevention of anemia would be a better strategy.
The desire to avoid the use of blood products coupled with the need to treat symptomatic anemia has led to trials of recombinant EPO in premature newborns. Low plasma EPO levels are a major contributing factor in the pathogenesis of anemia of prematurity. This is caused by reduced responsiveness of the liver compared to the kidney to tissue hypoxia and increased clearance of EPO in premature infants (1). When plated in clonogenic assays, erythroid progenitors from neonates demonstrate good responsiveness to EPO with an increase in BFU-E and CFU-E colonies (2,3). Furthermore, EPO in sufficient doses combined with adequate iron and protein supplements can induce an increase in the hemoglobin concentration in neonates. However, it remains unclear if these findings can be translated into a clinically meaningful reduction in the need for red blood cell (RBC) transfusions in premature neonates. In clinical trials reported to date, EPO has been started either early (within the first week of life) (47,48,49,50,51,52 and 53) or late (about 3 weeks of age) (34,36,54,55,56,57,58,59,60 and 61). In general, infants receiving larger EPO doses at both time points have shown improvement in reticulocyte counts and hemoglobin levels and a decrease in the number of transfusions per infant. Of importance, however, early initiation of EPO therapy was not associated with a significant reduction in the number of infants transfused, and in groups treated later most red blood cell exposures occurred before the start of EPO therapy.
In a recent meta-analysis Vamuakas and Strauss (62) analyzed 21 prospective controlled trials of EPO in newborn infants published during the period 1990 through 1999. The clinically relevant end points were a reduction in the number and volume of RBC transfusions. The investigators found that treatment with EPO reduced RBC transfusions by an average of 11ml/kg, a value that was statistically significant (p < 0.001). However, there was extreme variation among trials. The variability in the studies included birth weight of study subjects, postnatal age at study entry, frequency and dose of EPO, use of iron supplementation, and triggers for RBC transfusion. Unfortunately, even among studies in which these variables were similar there were differences in results.
In another meta-analysis by Garcia and associates (63) the authors analyzed 8 randomized placebo-controlled studies that administered EPO or placebo after the first week of life and focused on erythrocyte transfusion needs after the third week of life (“late” transfusions). In this specific group of patients they found that neonates in the EPO group received significantly fewer transfusions proportional to the dose of EPO administered. Based on these two analyses, it remains unclear whether EPO treatment should be universally recommended for all premature babies as a means to prevent clinically significant anemia that will require RBC transfusion. It is also unclear whether the cost of the RBC transfusions that are avoided by treatment with EPO is higher than the cost of EPO. Furthermore, although no adverse effects of EPO could be demonstrated by 1 year of age (53) the long term side effects of EPO in premature infants is unknown. Recently development of neutralizing antierythropoietin antibodies was demonstrated in patients with chronic renal failure who developed pure red cell aplasia following treatment with EPO. These patients were adults who received EPO treatment for 3 to 67 months. The condition resolved several months after cessation of EPO treatment during which time the patients required multiple RBC transfusions. Whether neonates who are treated with EPO for less than 3 months can develop neutralizing antibodies remains to be determined (64), and this potential complication of EPO therapy should be taken into consideration when assessing the risk:benefit of EPO therapy in newborn infants (65).
In summary, the overall incremental benefit of EPO therapy in neonates in the setting of rigorous transfusion guidelines and effective strategies to minimize iatrogenic (phlebotomy) blood losses, plus appropriate iron and protein supplementation is not clear and requires further study in carefully designed prospective clinical trials. Alternative transfusion strategies, discussed in detail later in this chapter, may be equally effective in preventing multiple donor exposure and may be more cost-effective. Currently, the most prudent approach is for individual neonatal units to assess the impact of newer transfusion strategies in their population before implementing routine EPO therapy (66).
If a decision is made to administer recombinant EPO to decrease RBC transfusions to ill extremely low birth weight infants (birth weight <1,000 g) Calhoun and colleagues have recommended that a dose of 200 U/kg/day of EPO plus iron supplementation be given for two weeks; if the goal is to decrease or possibly eliminate the need for late transfusions to very low birth weight infants (birth weight <1,500 g) with the anemia of prematurity or the late anemia of Rhesus hemolytic disease the investigators recommend giving 400 U/kg of EPO three times per week subcutaneously for 2 weeks with added iron (67).
Anemia Caused by Blood Loss
Blood loss resulting in anemia may occur prenatally, at the time of delivery, or postnatally. Blood loss may be the result of occult hemorrhage before birth, obstetric accidents, internal hemorrhages, or excessive blood sampling for diagnostic studies (Table 46-2). Faxelius and colleagues (68) associated a low erythrocyte volume with a maternal history of bleeding in the late third trimester, placenta previa, abruptio placentae, nonelective cesarean section, deliveries associated with cord compression, Apgar scores less than 6, an early central venous hematocrit less than 45%, and a mean arterial pressure less than 30 mm Hg.
Occult Hemorrhage Before Birth
Occult hemorrhage before birth may be caused by bleeding of the fetus into the maternal circulation or by the bleeding of one fetus into another in multiple pregnancies. In approximately 50% of all pregnancies, some fetal cells can be demonstrated in the maternal circulation (69). In about 8% of pregnancies, from 0.5 to 40.0 mL of blood is transferred from the fetus to the mother at birth, and in 1% of pregnancies, the blood loss exceeds 40 mL. Fetomaternal hemorrhages are more common after traumatic diagnostic amniocentesis or external cephalic version.
TABLE 46-2 TYPES OF HEMORRHAGE IN THE NEONATE | |
---|---|
|
Fetomaternal Hemorrhage
The clinical manifestations of a fetomaternal hemorrhage depend on the volume of the hemorrhage and the rapidity with which it has occurred. A sudden and unexpected decrease in fetal movements may be a warning sign of an acute, massive fetomaternal hemorrhage (70). The prognosis for such cases is poor and may be improved by prompt delivery and a neonatal transfusion, or if the fetus is premature by cord sampling and an intrauterine transfusion (71,72 and 73). If the hemorrhage has been prolonged or repeated during the course of the pregnancy anemia develops slowly giving the fetus an opportunity to develop hemodynamic compensation. These infants may manifest only pallor at birth. After acute hemorrhage just before delivery, the infant may be pale and sluggish, with gasping respirations and signs of circulatory shock.
The degree of anemia varies. Usually, the hemoglobin is less than 12.0 g/dL before the physician recognizes signs and symptoms of anemia. Hemoglobin values as low as 3.0 to 4.0 g/dL have been recorded in infants who were born alive and survived. If the hemorrhage has been acute, and particularly in hypovolemic shock, the hemoglobin value may not reflect the magnitude of the blood loss. Several hours may elapse before hemodilution occurs and the magnitude of the hemorrhage is appreciated. In general, a loss of 20% of the blood volume acutely is sufficient to produce signs of shock and is reflected in a fall in hemoglobin concentration within 3 hours of the event.
In acute and chronic hemorrhage, the erythrocytes usually appear normochromic and normocytic. Rarely in chronic hemorrhage, the cells appear hypochromic and microcytic, indicating fetal iron deficiency anemia (74).
If anemia is a direct result of a fetomaternal hemorrhage, the Coombs test is negative, and the infant is not jaundiced. Infants with anemia secondary to blood loss generally have lower than average bilirubin values throughout the neonatal period as a consequence of their reduced erythrocyte mass.
The diagnosis of a fetomaternal hemorrhage great enough to result in anemia at birth can be made with certainty only by the demonstration of fetal cells in the maternal circulation. The Kleihauer technique of acid elution is the simplest and most commonly employed method for the detection of fetal cells (75). The test is based on the property of HbF to resist elution from the cell in an acid medium. The acid elution technique can be relied on with certainty for diagnosis only when other conditions capable of producing elevations in maternal HbF levels are absent. These include maternal thalassemia minor, sickle-cell anemia, hereditary persistence of HbF, and in some normal women, a pregnancy-induced rise in HbF production (76). In these conditions, the appearance of the Kleihauer test, with many cells containing variable amounts of HbF, is easily differentiated from that of a true transplacental hemorrhage, in which the fetal cells containing high concentrations of HbF are readily differentiated from the maternal cells containing no HbF.
The diagnosis of a fetomaternal hemorrhage may be missed in situations in which the mother and infant are incompatible in the ABO blood group system. In such instances, the infant’s A or B cells are rapidly cleared from the maternal circulation by the maternal anti-A or anti-B and may not be seen in the Kleihauer preparation.
Twin-to-twin Transfusion
Twin-to-twin transfusion is observed in 13% to 33% of monozygotic multiple births with monochorial placentas (77). In approximately 70% of monozygotic twin pregnancies, a monochorial placenta exists. Blood exchange between twins may produce anemia in the donor and polycythemia in the recipient. If a significant hemorrhage has occurred, the difference in hemoglobin between the twins exceeds 5.0 g/dL. There is a maximal discrepancy of 3.3 g/dL in cord blood hemoglobin concentration in dizygotic twins. The anemic twin may develop congestive heart failure and hydrops, and the plethoric twin may manifest symptoms and signs of the hyperviscosity syndrome, disseminated intravascular coagulation (DIC) and hyperbilirubinemia.
The hemorrhage may be acute or chronic. Tan and associates (77), on the basis of a review of 482 twin pairs in which 35 were found to have the transfusion syndrome, pointed out how the difference in weight of the twins could be used to establish the timing of the hemorrhage. If the weight difference exceeded 20% of the weight of the larger twin, the transfusion was chronic, and the smaller infant was invariably the donor. The anemic, smaller twin displayed reticulocytosis. If the difference in the weight of the twins did not exceed 20% of the weight of the larger twin, the larger twin was the donor in almost 50% of cases. In these presumably acute transfusions around the time of birth, significant reticulocytosis was not observed in the anemic donor.
If twin-to-twin transfusion is suspected, attempts to confirm it by placental examination should be made. The placentas of all multiple pregnancies should be routinely examined for purposes of genetic counseling. If hematologic evidence has not been obtained, and the infants have died, other findings may suggest the diagnosis, including polyhydramnios of the recipient’s amniotic sac and oligohydramnios of the donor and marked differences in the size and organ weights of the twins.
With the advent of accurate ultrasound assessment of the fetus, the diagnosis of twin-to-twin transfusion in utero has become possible, and a careful assessment of chorionicity in twins undergoing first trimester ultrasound scanning is recommended (78). The early detection of monochorionic twins identifies a high-risk pregnancy that should be managed in obstetric centers experienced in dealing with such cases. In cases of severe twin to twin transfusion, the donor (anemic) twin is smaller, and there is associated oligohydramnios; the recipient (polycythemic, hypervolemic) twin is larger, and there is associated polyhydramnios. Intrauterine diagnosis is therefore dependent on identification of same sex, size difference, oligohydramnios/polyhydramnios, and a monochorionic placenta. When diagnosed in utero, twin-to-twin transfusion syndrome can be classified into five discreet stages (79). Stage I is defined by the finding of isolated discrepancy in amniotic fluid volumes between fetuses; absence of a urine filled bladder in the donor fetus defines Stage II, absent or reversed end-diastolic flow in the umbilical artery of the donor fetus or abnormal venous Doppler pattern in the recipient, such as reversed flow in the ductus venosus or pulsatile umbilical venous flow Stage III, hydrops fetalis Stage IV and demise of one or both fetuses Stage V. Perinatal outcomes correlate with disease severity as assessed by the stage at presentation and gestational age at delivery; overall the perinatal mortality rate for the twin-to-twin transfusion syndrome is 30% to 50% (80). Therapy has included repeated amniocentesis to reduce polyhydramnios, photocoagulation of placental vascular anastomoses, amniotic septostomy, and selected feticide by cord occlusion (78).
Obstetric Accidents and Complications
Obstetric accidents and malformations of the placenta and cord may be responsible for major blood loss at the time of delivery. These accidents may be unreported to the pediatrician and may result in diagnostic confusion about the cause of shock in the early hours of life or the presence of pallor and unexplained anemia during the second or third day of life.
The obstetric conditions that can produce neonatal hemorrhage are listed in Table 46-2. Severe and often fatal fetal hemorrhage may accompany placenta previa, abruptio placentae, or accidental incision of the placenta or umbilical cord during a cesarean section. A tight nuchal cord may cause venous obstruction leading to excessive blood trapping in the placenta and resulting in severe hypovolemia (81) and anemia (82). A prospective study of red cell mass suggested that babies born with a tight nuchal cord had a significantly lower red cell mass than controls (83).
In women with late-third-trimester bleeding, Clayton and associates (84) were able to anticipate the birth of a possible anemic infant by examining the vaginal blood for the presence of fetal erythrocytes, employing the acid elution technique of Kleihauer (75,84).
It is good pediatric practice to obtain a hemoglobin measurement routinely at the time of delivery of all babies born of women with late third-trimester bleeding. This determination should be repeated in 6 to 12 hours to observe the expected fall in hemoglobin resulting from the hemodilution that follows recent blood loss.
Severe bleeding as a result of an obstetric accident or complication of delivery often results in the birth of a pale, limp infant. Respirations, which usually commence spontaneously, are often irregular and gasping. They are not associated with retraction, as in conditions accompanied by primary pulmonary disease. Cyanosis is minimal, and the infant’s pale color is not improved by oxygen administration. The peripheral pulses are weak or absent, and the blood pressure is reduced. The venous pressure measured after the insertion of an umbilical catheter is found to be extremely low.
Internal Hemorrhage
Anemia that appears in the first 24 to 72 hours of life and is not associated with significant jaundice is commonly caused by hemorrhage at the time of birth or by a postnatal internal hemorrhage. Traumatic deliveries may result in subdural or subarachnoid hemorrhages or cephalhematomas of sufficient magnitude to produce anemia. Subaponeurotic or subgaleal hemorrhages are relatively common after vacuum extraction and may lead to significant neonatal anemia.
Breech deliveries may be associated with hemorrhage into the adrenals, kidney, spleen, or retroperitoneal area. Rupture of the liver or subcapsular hemorrhage into the liver may occur more commonly than is clinically recognized (85,86 and 87). An infant with a ruptured liver may appear well for the first 24 to 48 hours of life and then suddenly go into shock. The abdomen may appear distended, and a mass contiguous with the liver is often palpable. Shifting dullness on abdominal percussion can often be demonstrated, and an elevation of the right hemidiaphragm may be seen on the radiograph. Splenic rupture may occur after a difficult delivery or as a result of the extreme distension of the spleen that is often seen in babies with severe erythroblastosis fetalis. The physician should always suspect a rupture of the spleen when an anemic, and often hydropic, infant with erythroblastosis is found to have a low initial venous pressure at the time of exchange transfusion. The diagnosis of intraabdominal hemorrhage is readily made with ultrasonography.
In infants with birth weights less than 1,500 g, bleeding into the cerebral ventricles, subarachnoid space, and parenchyma can also produce significant decreases in hemoglobin concentration.
Iatrogenic Anemia due to Blood Sampling
Anemia appearing during the first week of life is often caused by blood removal for diagnostic studies required for the frequent monitoring of critically ill infants. Removal of more than 20% of a subject’s blood volume produces anemia. In an infant of 1,500 g, this represents a blood loss of only 25 mL. If frequent blood sampling is necessary, a flow sheet should be used to record the amount removed at any given time. This simple technique often converts a diagnosis of idiopathic anemia to one of iatrogenic anemia.
Despite the use of micromethods using small volumes of blood by most laboratories, cumulative blood losses through sampling for laboratory monitoring are often surprisingly large in small infants. Blanchette and Zipursky (88) reported an average blood loss of 22.9 mL of packed cells from 59 premature infants studied through the first 6 weeks of life. Forty-six % (26 of 57) of the infants studied had cumulative losses that exceeded their circulating erythrocyte mass at birth (Fig. 46-3); in a few cases, losses were equivalent to two or three times the infants’ initial circulating erythrocyte masses. Because these losses must be replaced, at least in part, by erythrocyte transfusions, some infants had the equivalent of a double- or triple-volume exchange transfusion simply as a result of blood sampling for laboratory tests. Approximately 10% of all blood loss during sampling for laboratory monitoring was hidden and represented blood on cotton swabs or in the dead space of syringes or tubing of butterfly sets used to collect blood samples (89).
There is a strong correlation between the volume of blood sampled and that transfused (Fig. 46-4), suggesting that much of the erythrocyte transfusion requirements of ill, premature infants is a direct consequence of blood loss for essential laboratory monitoring (88). In the study by Blanchette and Zipursky (88), significantly more blood was sampled from infants judged to be clinically ill than from healthy premature infants. Iatrogenic blood loss through sampling was significant in both groups (mean ± 1 SD = 26.9 ± 9 and 14.6 ± 5 mL, respectively). These comparative volumes may not appear large, but the actual values must be compared with a total erythrocyte mass that varies between 32.3 and 45.5 mL/kg (90,91,92,93 and 94). The removal of 1 mL of blood from a 1-kg infant is equivalent to removing 70 mL of blood from an average adult, and it is therefore not surprising that repeated blood sampling, even with capillary samples, can have a profound effect on the hemoglobin concentration of small, premature infants. Ballin and colleagues have demonstrated that, if the erythrocytes that are discarded from samples drawn in which only the plasma was used were instead reinfused, the fall in hemoglobin concentration could be substantially reduced (95).
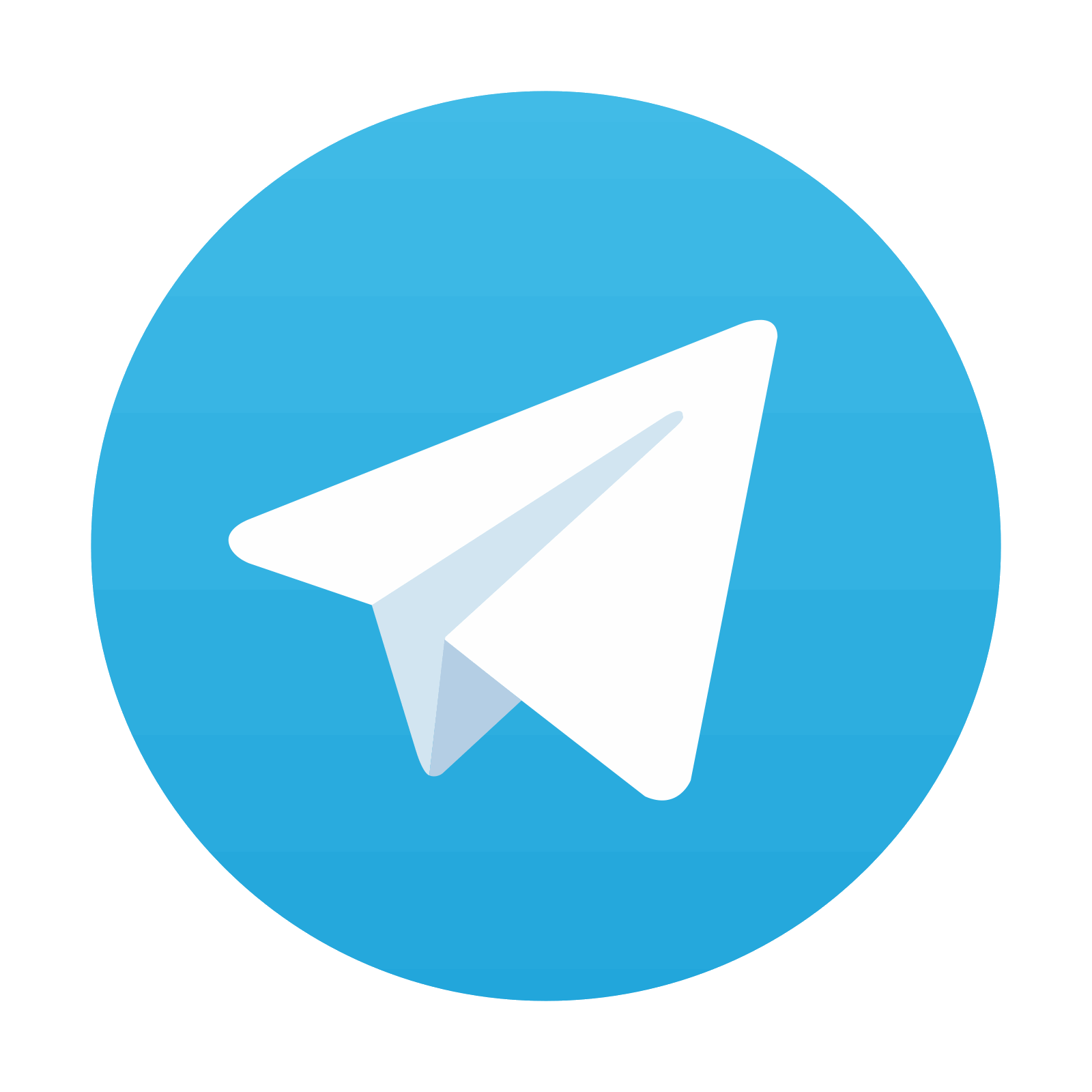
Stay updated, free articles. Join our Telegram channel
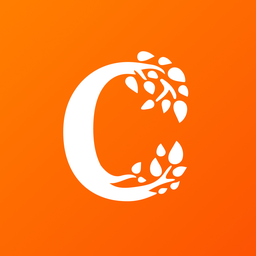
Full access? Get Clinical Tree
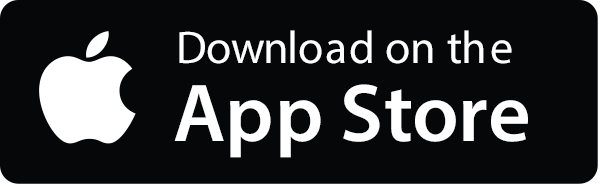
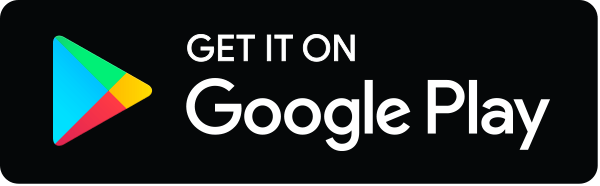