Since phenytoin was introduced for the treatment of epilepsy by Merritt and Putnam in 1938, it has become one of the most widely used and extensively investigated antiepileptic drugs. It has been administered to patients of all ages and thus provides a good model for evaluating the effects of age on pharmacokinetics. In addition, phenytoin is unique among the commonly prescribed antiepileptic drugs because of its nonlinear elimination kinetics (Figure 60.1) (1,2).
CHEMISTRY
Phenytoin is a weak acid, having a pKa of 8.06 (3) (Table 60.1). Phenytoin sodium is approximately 92% phenytoin, a difference that is sometimes important because of phenytoin’s nonlinear elimination kinetics. Phenytoin has limited aqueous solubility, approximately 20 mg/L, unless solubilizing agents are added to the solution. Parenteral formulations of phenytoin are strongly basic and contain the alcohols propylene glycol and ethanol. These formulations are potentially cardiotoxic and thus should be administered slowly intravenously to avoid bradyarrhythmia and hypotension. The phenytoin prodrug fosphenytoin has enhanced solubility and circumvents this hazard; although it is considerably safer for intravenous (IV) administration, it is also considerably more expensive.
CLINICAL EFFICACY
The spectrum of activity of phenytoin includes status epilepticus, partial seizures, partial seizures secondarily generalized, tonic seizures, and generalized tonic–clonic seizures (4,5). Increasing doses and levels of phenytoin are associated with progressive reduction of seizures in adults (6) and in children (7). When administered following head trauma, phenytoin reduces the risk of seizures within the initial 7 days, but it has no effect on the long-term development of epilepsy (8). A long mainstay in the treatment of status epilepticus, phenytoin has been displaced as the drug to use first by lorazepan (9).
Although the therapeutic range is widely quoted at 10 to 20 mg/L, individual patients may require lower or higher levels for successful treatment. Phenytoin is not effective against generalized absence seizures and may increase their frequency; it is also ineffective in preventing recurrent febrile seizures. Phenytoin has been widely used to treat neonatal seizures, including neonatal status epilepticus (10–13). After phenobarbital fails to control neonatal seizures, the chance that phenytoin will succeed is approximately 50% (14,15). The response of neonatal seizures caused by hypoxia, ischemia, or hemorrhage is poor (15). Phenytoin is also used to treat kinesigenic choreoathetosis (16).
PHENYTOIN ADMINISTRATION
Phenytoin is available for parenteral and oral administration (Table 60.1). Although IV phenytoin is effective in stopping status epilepticus and in preventing recurrent seizures, it is slower acting than a benzodiazepine in stopping acute convulsions (17). However, when status has been interrupted by a short-acting drug such as diazepam, phenytoin can be administered next to prevent seizure recurrence. When phenytoin is administered intravenously, it must be given slowly to reduce the risk of cardiovascular toxicity (Table 60.2). For this reason, the safer formulation fosphenytoin is preferred because of the desirability of stopping status epilepticus as rapidly as possible.
Phenytoin has several advantages in the acute treatment of seizures. It is not sedating and thus does not potentiate sedation and respiratory depresion as do benzodiazepines and barbiturates. When seizures persist after administration of phenytoin in status, cumulative doses of up to 30 mg/kg can be given initially but should not be exceeded unless it is documented that phenytoin levels are not excessive, because high phenytoin levels can cause seizures. Intramuscular phenytoin is not effective in the acute treatment of seizures.
In chronic oral therapy, phenytoin usually can be administered twice daily. Because of the nonlinear kinetics of phenytoin, it is best to begin with a low average dose and increase the dose stepwise until the desired clinical result is obtained. In young children, initial doses of 8 to 10 mg/kg/day are reasonable, whereas older children, adolescents, and young adults should begin with 6 to 8 mg/kg/day (18). As the dose is raised, progressively smaller increases should be made to avoid disproportionate increases in phenytoin level and resultant toxicity. If precise adjustment of phenytoin levels is necessary, the distinction between phenytoin and phenytoin sodium becomes an important consideration when mixing or switching to different products that contain phenytoin. The concentration of phenytoin in contemporary suspensions remains fairly uniform, but, in the past, suspensions were ill advised because of the tendency for phenytoin to settle out. Doses from the top of the bottle were lower than expected, whereas those from the bottom were higher than expected, because the suspended medication settled at the bottom after standing. In all instances when phenytoin dose has been changed, patients should be reexamined to evaluate whether the dose is correct because of the unpredictable relationship between phenytoin dose and concentration.
FIGURE 60.1 Dose versus concentration curve for drugs with nonlinear elimination kinetics.
Source: From Ref. (2). Glazko AJ. Phenytoin: chemistry and methods of determination. In: Levy RH, Mattson RH, Meldrum BS, Penry JK, eds. Antiepileptic Drugs. 3rd ed. New York, NY: Raven Press; 1989:159–176. Reproduced with permission.
TABLE 60.1
PHENYTOIN REFERENCE INFORMATION | |
Molecular weight of sodium phenytoin |
274.25 |
Molecular weight of phenytoin |
252.26 |
pKa of phenytoin |
8.06 |
Conversion factor |
CF = 1000/252.3 = 3.96 |
Conversion |
μg/mL (or mg/L) × 3.96 = μmoles/L |
Formulations |
|
Phenytoin suspension (Dilantin) |
50 mg/mL |
Phenytoin (Dilantin Infatabs) |
50 mg |
Phenytoin sodium (Dilantin capseals) |
30, 60, or 100 mg |
Type of elimination kinetics |
Nonlinear |
TABLE 60.2
ASPECTS OF PHENYTOIN ADMINISTRATION | |
Intravenous Dose |
15–20 mg/kg* |
Intravenous administration rates |
|
Rate in adults |
<50 mg/min |
Rate in infants and children |
<3 mg/kg/min |
Maintenance dose |
6–20 mg/kg/day |
Therapeutic concentration range |
10–20 mg/L |
*Administer slowly to avoid cardiovascular toxicity.
BIOTRANSFORMATION, PHARMACOKINETICS, AND INTERACTIONS IN HUMANS
Phenytoin is largely eliminated by enzymatic biotransformation in the liver to 5-parahydroxyphenyl, 5- phenylhydantoin (HPPH), which is the major metabolite, and to a dihydrodiol that accounts for approximately 10% of phenytoin metabolites in urine. Both of these metabolites are thought to be derived from a highly reactive arene oxide (epoxide) intermediate (15,19). Negligible amounts of unmetabolized phenytoin are excreted in urine.
Phenytoin is metabolized by the cytochrome P450 enzymes CYP2C9 and CYP2C19 (20,21). In some families that metabolize phenytoin slowly, mutations have been identified that account for these pharmacokinetic differences (22). Odani et al identified a mutation of CYP2C9 caused by a substitution of leucine for isoleucine at position 359 that results in a reduction of 33% in maximal capacity to metabolize (Vmax) phenytoin among Japanese people. Mutations in CYP2C19 reduced Vmax by as much as 14% (21). Chung et al have confirmed CYP2C variants in samples from Taiwan and Malaysia, in addition to Japan (23).
Phenytoin has nonlinear kinetics in all age groups (1,24–31). As the dose and concentration of phenytoin increase, the eliminating mechanism becomes progressively saturated, reducing the fraction of phenytoin that is eliminated per unit of time. This leads to a nonlinear relationship between phenytoin dose and concentration (Figure 60.1). Because increasing doses cause disproportional rises in the phenytoin level, smaller increments in dose should be made as the phenytoin level approaches the therapeutic range. Phenytoin kinetics are best characterized by Michaelis–Menten equations. However, the concept of half-life is widely used in clinical pharmacokinetics and therefore merits discussion (Table 60.3).
PHENYTOIN HALF-LIFE
The kinetics of phenytoin elimination are concentration- dependent. Although the half-life of phenytoin is widely quoted and discussed, applying the concept of a half-life to phenytoin kinetics is technically improper, because phenytoin does not have first-order elimination kinetics. The apparent half-life (t50%) of phenytoin changes depending on the concentration range over which it is measured. As a consequence of phenytoin’s nonlinear elimination kinetics, increasing concentrations lead to prolongation of the apparent half-life. When phenytoin levels are high, the apparent half-lives can be quite prolonged (26,31). As the level declines, the rate of phenytoin elimination accelerates and the apparent half-life becomes shorter (Figure 60.2). Thus, at different concentrations, different half-lives can be measured in a single patient. The apparent half-life is sometimes designated by 150%. The t50% is directly related to the concentration at which the t50% is determined (Figure 60.3).
TABLE 60.3
PHENYTOIN PHARMACOKINETICS | |
Protein binding |
90% |
Volume of distribution in newborns |
0.8–1.2 L/kg |
Older children and adults |
0.7–0.9 L/kg |
Half-life |
Concentration-dependent |
Nonlinear kinetic parameters |
|
KM |
5 mg/L |
Vmax |
10–20 mg/kg/day |
FIGURE 60.2 Log phenytoin concentration versus time curve that was obtained in a 14-month-old who ingested a large dose of phenytoin. Note that the observed half-life becomes progressively shorter as the concentration declines.
Source: From Ref. (18). Browne TR, LeDuc B. Phenytoin: chemistry and biotransformation. In: Levy RH, Mattson RH, Meldrum BS, eds. Antiepileptic Drugs. 4th ed. New York, NY: Raven Press; 1995:283–300. Reproduced with permission.
Because the t50% of phenytoin varies, it takes longer than expected for patients to reach a steady state. This is particularly a problem when patients take relatively high doses that are close to their maximal phenytoin eliminating capacity (Vmax). As a rule of thumb, at least 2 weeks should be allowed before phenytoin levels are considered to be at steady state after the dose is changed. In some patients who have very high levels, even more time may be required.
FIGURE 60.3 Relationship of the initial concentration (Ci) to the apparent half-life that was observed in an infant taking phenytoin. As the concentration increased, the apparent half-life (t50%) was prolonged.
The changing t50% of phenytoin is occasionally useful in clinical situations. For example, small increases in the average phenytoin level can be achieved by giving the total daily dose as a single dose (32). This increases the average level slightly because the large single dose produces a higher initial phenytoin concentration, thereby prolonging the t50%. Dividing the daily dose into more frequent daily doses has the opposite effect and slightly lowers the average concentration.
NONLINEAR KINETICS, KM AND VMAX
Phenytoin kinetics are best characterized by Michaelis–Menten kinetic parameters KM and Vmax. Children and adults have similar KM values of approximately 5 mg/L. However, children have higher capacity to eliminate phenytoin than adults, resulting in higher Vmax (2,24,25,33). Much of the variation in KM is caused by drug interactions.
There are several methods for calculating the KM and Vmax. The easiest and most reliable method is the direct linear plot (34). This is a graphic solution that can be performed at the bedside. The method depends on knowing the patient’s phenytoin level at two or more pairs of doses and steady-state concentrations. The concentrations should be obtained at consistent times after doses. When phenytoin is given twice daily, the best times to measure the level are just prior to a dose and 8 hours after a dose (35). Once KM and Vmax are known, it is possible to estimate the relationship between future doses and concentrations.
The direct linear plot technique for calculating KM and Vmax is fairly simple and is illustrated in Figure 60.4 (36). The negative value of the phenytoin level is plotted on the horizontal axis and the dose is plotted on the vertical axis. The dose and level pairs are connected by lines that are extended up and to the right, where they intersect. This point of intersection is used as a fulcrum for determining KM, Vmax, and other dose-level relationships. A horizontal line drawn from the point of intersection to the vertical axis is Vmax. A vertical line drawn from the intersection indicates KM on the horizontal axis. The relationships between other doses and levels are solved by drawing additional lines from the point of intersection as shown in Figure 60.4.
There are many nomograms and related methods for predicting the relationship between phenytoin dose and concentration in various groups of patients (30). Although these provide heuristic exercises in clinical pharmacology, they are untrustworthy guides to phenytoin dosing. In one study, significant errors in predicted levels occurred in 21% to 38% of subjects (37). Large errors are most likely among patients who need high phenytoin levels. For this reason, every patient whose phenytoin dose is changed should be reevaluated after an appropriate interval (30). Although nomograms can reduce trial and error in estimating phenytoin doses, they are no substitute for patient follow-up.
FIGURE 60.4 Direct linear plot. In this example KM = 4 mg/L and Vmax = 12 mg/kg/day. Predicted values are indicated by the dotted line. Insert indicates the shape of the dose versus concentration curve. See text for details.
Source: From Ref. (36). Chan E. Single-point phenytoin dosage predictions in Singapore Chinese. J Clin Pharmacy Ther. 1997;22:47–52. Reprinted with permission from John Wiley & Sons.
PHENYTOIN ABSORPTION
The nonlinear kinetics of phenytoin can affect the apparent extent of phenytoin absorption (bioavailability). Slowing the absorption rate has the practical result of reducing the apparent bioavailabililty (38). Computer simulations indicate that, at average values of KM and Vmax, increasing the absorption half-life from 0 to 1.2 hours causes the apparent bioavailability to decrease by approximately 25% (39). If the actual fraction that is absorbed also decreases slightly, the reduction in apparent bioavailability is greater. For example, with 90% absorption and an absorption half-life of 0.9 hours, the apparent bioavailability declines to 69%.
Although phenytoin is well absorbed after oral administration, the rate of absorption depends on the formulation. Changing excipients has been associated with changes in steady-state levels (40). But, even without changes in excipients, other aspects of tablet formulation can affect the absorption rate. Thus, switching formulations can change the phenytoin levels significantly. For this reason, generic substitution of phenytoin should be discouraged.
In infants, peak phenytoin concentrations occur 2 to 6 hours after oral dosing (41). In older children, peak concentrations occur 3 to 10 hours following oral doses. The administration of phenytoin with food further delays the time to peak concentrations in patients of all ages (42). However, phenytoin taken postprandially produces 40% higher concentrations than when it is taken before meals. Thus, the relationship between phenytoin doses and meals should be consistent.
Intramuscular administration of phenytoin is associated with slow and erratic absorption (43). Because of the low solubility of phenytoin, it can crystallize and precipitate at the injection site. Although phenytoin absorption is eventually complete, intramuscular administration of phenytoin is unreliable and should be avoided (44). If intramuscular administration is needed, fosphenytoin should be given.
PHENYTOIN DRUG-PROTEIN BINDING
The unbound drug concentration, not the total drug concentration, determines the drug’s action. Phenytoin is normally 90% bound to constituents of serum, mainly to albumin. In diseases with hypoalbuminemia, such as renal disease, hepatic disease, severe malnutrition, burns, and pregnancy (45,46), unbound phenytoin levels increase such that the total phenytoin levels no longer provide reliable indicators of the unbound levels. In these situations, measuring the unbound phenytoin level is sometimes indicated.
Phenytoin binding is also reduced by bilirubin and acidic compounds such as fatty acids, including valproic acid, and salicylate, which displace phenytoin from protein binding (47). Among developmentally disabled patients, hypoalbuminemia and comedication with valproate have been associated with unbound phenytoin fractions as high as 17% (48). When the unbound phenytoin fraction increases chronically, hepatic metabolism compensates such that the unbound concentration is readjusted to the previous steady-state level. This leads to a decline in the total phenytoin level, but the effect on unbound levels is insignificant. Thus, chronic changes in binding have a negligible effect on unbound phenytoin concentrations unless the dose is changed (49). On the other hand, acute changes in phenytoin binding such as those associated with dialysis may cause transient and symptomatic changes in unbound phenytoin levels. Rapidly fluctuating valproate levels can increase unbound phenytoin levels transiently, resulting in transient phenytoin toxicity (50).
EFFECT OF AGE ON PHENYTOIN PHARMACOKINETICS
The maximal capacity to eliminate phenytoin is affected by genetic factors, age, sex, and drug interactions (51,52). However, it is important to note that the nonlinear kinetics of phenytoin have confounded most clinical investigations of the effect of age on phenytoin kinetics. When linear kinetic parameters such as half-life or relative clearance are used, the nonlinear kinetics often obscure age-related pharmacokinetic differences (26,53). In a practical sense, the nonlinear kinetics of phenytoin influence relative dosage requirements more than does age.
Newborns
Newborns that are exposed to phenytoin in utero usually eliminate transplacentally acquired phenytoin at rates that are comparable to those in adults. Studies of urinary metabolites indicate that these newborns rapidly metabolize phenytoin (54). On the other hand, newborns with seizures eliminate phenytoin slowly at first, but later, during the neonatal period, they eliminate it rapidly after their drug-eliminating mechanisms have matured (26).
Among all age groups, premature and full-term newborns who were not exposed in utero to inducing agents have the lowest relative capacity to eliminate phenytoin; thus, they require the lowest doses on average. Phenytoin concentrations and apparent half-lives vary extensively in newborns with seizures, more so than in any other age group. Newborns with seizures have several factors that act simultaneously to produce unstable phenytoin levels. These include nonlinear phenytoin kinetics, postnatal maturation of hepatic function, induction of phenytoin elimination by phenobarbital and other drugs, and slowed phenytoin absorption, which occurs after the newborns have been switched from intravenous to oral dosing. Most newborns who receive phenytoin have been treated previously with phenobarbital. Phenobarbital affects the hepatic biotransformation of phenytoin, increasing both Vmax and KM (2,55). For this reason, the changing phenytoin kinetics during the neonatal period preclude the use of steady-state methods for analyzing the nonlinear kinetics of phenytoin. These changes make it necessary to increase the phenytoin dose during the neonatal period if consistently high phenytoin levels are needed.
High phenytoin concentrations occur after intravenous loading doses, often leading to very long apparent half-lives in the first and second week of life. The phenytoin half-lives that have been reported in newborns vary widely, with extremes of 6 hours to more than 200 hours after intravenous therapy (11,26,56). Bourgeois and Dodson (26) found that the phenytoin t50% ranged from 6.9 to 140 hours, with an average value of 57.3 ± 48.2 hours. In newborns aged 3 to 5 weeks, the t50% decreased by two-thirds.
After the first week of life, shorter phenytoin half-lives have been reported, but some newborns continue to have prolonged half-lives if phenytoin levels are high (11,50). Painter et al (11,12) reported an average value of 104 ± 17 hours during the second week of life. In a different group of 16 newborns with seizures, half-lives diminished considerably after the first week of life (26). Among children more than 1 week old, there was good correlation (r = 0.88 to 0.93) between the apparent half-life and the initial phenytoin concentration (27). Correcting for initial concentration revealed that newborns ages 3−5 weeks had the shortest half-life, averaging 19.7 and 12 hours at initial phenytoin concentrations of 18 and 10 mg/L, respectively.
After the first or second week of life, the capacity for phenytoin elimination increases and phenytoin concentrations often decline. Older newborns and young infants require doses as high as 18 to 20 mg/kg/day to achieve therapeutic levels (4). If declining concentrations occur when phenytoin administration is switched from the intravenous to the oral route, the changing dose requirements give the appearance of malabsorption of phenytoin (11,12).
Newborns absorb phenytoin slowly but completely after oral administration, with less than 3% of administered phenytoin found in feces (57). Studies using stable isotope-labeled phenytoin also indicate complete absorption (58).
Similarly, comparisons of levels obtained after intravenous and oral doses indicate complete absorption of orally administered suspensions (59). Thus, the decline in phenytoin levels that takes place in the second or third week of life is caused by an increasing capacity for phenytoin biotransformation plus the unique consequences of nonlinear elimination kinetics. Despite numerous statements to the contrary, newborns do not malabsorb phenytoin. Reliable concentrations can be produced by oral administration even in premature newborns (59).
It is technically difficult to actually measure phenytoin bioavailability in newborns because of the changing elimination kinetics during the newborn period. For this reason, computer simulations have been used to provide insight into the problem. These simulations indicate that the changing phenytoin elimination kinetics (Vmax and KM)—and the slower absorption rates that occur after phenytoin administration is switched from intravenous to oral routes—both affect the phenytoin level (40,41). For example, slowing the absorption rate of phenytoin decreases the apparent bioavailabililty by as much as 26%.
The effects of varying the absorption rate and KM on the apparent extent of phenytoin absorption are modest compared to the large changes that occur when Vmax increases. Based on computer simulations, increasing Vmax is expected to produce sizeable reductions in apparent bioavailability even when absorption is complete (39). For example, increasing Vmax three-fold from 5 mg/kg/day to 15 mg/kg/day causes the apparent bioavailability to decrease by 77%. Variations in KM cause smaller changes in apparent bioavailability; changing KM from 5 mg/L to 1 mg/L decreases the apparent bioavailability to 67%. Increasing KM from 5 to 10 mg/L, such as might occur because of an interaction with phenobarbital, has the opposite effect.
Infants and Children
Among all age groups, infants have the highest relative capacity to eliminate phenytoin, causing them to have average dosage requirements that are four-fold greater than adults. Vmax, but not KM, changes with increasing age (2,25) (Figure 60.5). After phenytoin-eliminating capacity peaks during infancy, it declines during childhood to adult values (24). In one study, the Vmax in infants ranged from 11 to 30 mg/kg/day and averaged 17.9 mg/kg/day (2). The average values in older children and adults are approximately 8 to 10 mg/kg/day in various studies. Although most of the variation in KM is caused by drug interactions, KM does vary independently as well. In one study, 28% of children had a KM of 2.5 mg/L or less (2). This low value of KM makes it technically difficult to adjust phenytoin levels when levels are greater than 10 mg/L because the dose versus concentration curve becomes progressively steep and nonlinear (Figure 60.6).
The higher relative clearance for phenytoin in children gradually declines until adult values are reached around ages 10 to 15 (see Figure 60.6). During this period, changes in body weight are offset by declining relative drug clearance, such that dosage changes are less frequent than expected. However, within any age group, individual patients deviate significantly from the group average. There are several causes for this variation: foremost among these are drug interactions and intercurrent illness.
FIGURE 60.5 Relationship between age and Vmax for phenytoin elimination.
Source: From Ref. (2). Glazko AJ. Phenytoin: chemistry and methods of determination. In: Levy RH, Mattson RH, Meldrum BS, Penry JK, eds. Antiepileptic Drugs. 3rd ed. New York, NY: Raven Press; 1989:159–176. Reproduced with permission.
FIGURE 60.6 Dose versus concentration curves for varying values of KM when Vmax is held constant at 15 mg/kg/day. Note that, as the values for KM decline from 10 to 2 mg/L, the curve becomes more nearly vertical through the concentration range of 10 to 20 mg/L. (A) KM = 10 mg/L; (B) KM = 5 mg/L; (C) KM = 2 mg/L.
Adolescents
No major changes in phenytoin kinetics have been described in adolescence. However, phenytoin concentrations fluctuate during the menstrual cycle. Although these fluctuations are usually modest, concentrations are higher at midcycle, when ovulation occurs, than at the time of menstruation. This suggests that increased concentrations of estrogen and progesterone interfere with phenytoin biotransformation (57). The fluctuations in phenytoin levels are most extensive in patients who have the highest concentrations.
DRUG INTERACTIONS AND NONLINEAR KINETICS OF PHENYTOIN
Phenytoin is both a cause and a casualty of pharmacokinetic drug interactions. First, it is a potent inducer of many enzymes in the cytochrome P450 drug-metabolizing system (60). By this mechanism, the addition of phenytoin increases the clearance and decreases the concentrations of most other antiepileptics that are eliminated by hepatic metabolism. Examples include carbamazepine, methsuximide, primidone, valproate, and most of the antiepileptic drugs that have been introduced in the 1990s, including felbamate, topiramate, and lamotrigine. Besides enhancing the clearance of these compounds, phenytoin also stimulates the clearance of steroids, including oral contraceptives, and the clearance of vitamins, including vitamin D, folic acid, and vitamin K (60,61).
Phenytoin elimination is extremely vulnerable to drug interactions by virtue of its dependence on hepatic metabolism for elimination and its nonlinear kinetics (62). Even though general trends can be described, the direction and extent of these interactions are highly unpredictable. For this reason, diligent clinical follow-up is required whenever a comedication is added to or deleted from treatment regimens that include phenytoin.
Phenobarbital consistently increases both the Vmax and KM of phenytoin elimination (2). Thus, phenobarbital has conflicting effects, acting simultaneously as an inducer and as a competitive inhibitor of phenytoin elimination. In groups of patients, the addition of phenobarbital to phenytoin regimens produces no change in the average phenytoin level, but, in individual patients, phenytoin levels can change significantly.
Drug interactions can make the adjustment of phenytoin easier or more difficult, depending on the drug that is involved. The phenobarbital–phenytoin interaction facilitates the titration of phenytoin levels in the upper part of the therapeutic range (see Figure 60.5). This pharmacokinetic benefit is due to the increased value of KM for phenytoin elimination, which leads to a mild degree of flattening of the phenytoin dose versus concentration curve. As a result, the dose–concentration relationship becomes more linear and more predictable. Carbamazepine interacts with phenytoin elimination by inducing cytochrome P450 enzymes CYP2C9 and CYP219 (20,63,64). The net effect of this interaction is to reduce both the KM and Vmax for phenytoin elimination (2), which is a complex interaction that usually leads to a reduction in phenytoin level. This interaction makes readjustment of phenytoin levels technically difficult because of the reduced KM value and increased nonlinearity of the dose–concentration relationship. Herbal treatment with Ginkgo biloba also induces CYP2C9 and has been reported to lower phenytoin levels (65). Valproate interacts with phenytoin by displacing it from binding sites on albumin and increasing the unbound phenytoin fraction. Valproate reduces the KM of phenytoin elimination but has no effect on Vmax (2,66). However, switching from the standard formulation of sodium valproate to a slow-release formulation can cause the phenytoin level to increase. Suzuki et al (66) found that the average phenytoin level went from 14.4 to 18.7 mg/L when the valproate formulation was switched to the slow-release formulation.
Certain drug interactions usually increase the phenytoin level. Chloramphenicol consistently inhibits phenytoin elimination, thus increasing phenytoin levels (67). Conversely, discontinuing chloramphenicol causes phenytoin levels to decline dramatically. Isoniazide also usually increases phenytoin levels (68). According to the Boston Collaborative Drug Surveillance Program, 27% of the patients who take isoniazide plus phenytoin develop phenytoin toxicity if the phenytoin dose is not adjusted (69).
EFFECT OF ILLNESS ON PHENYTOIN PHARMACOKINETICS
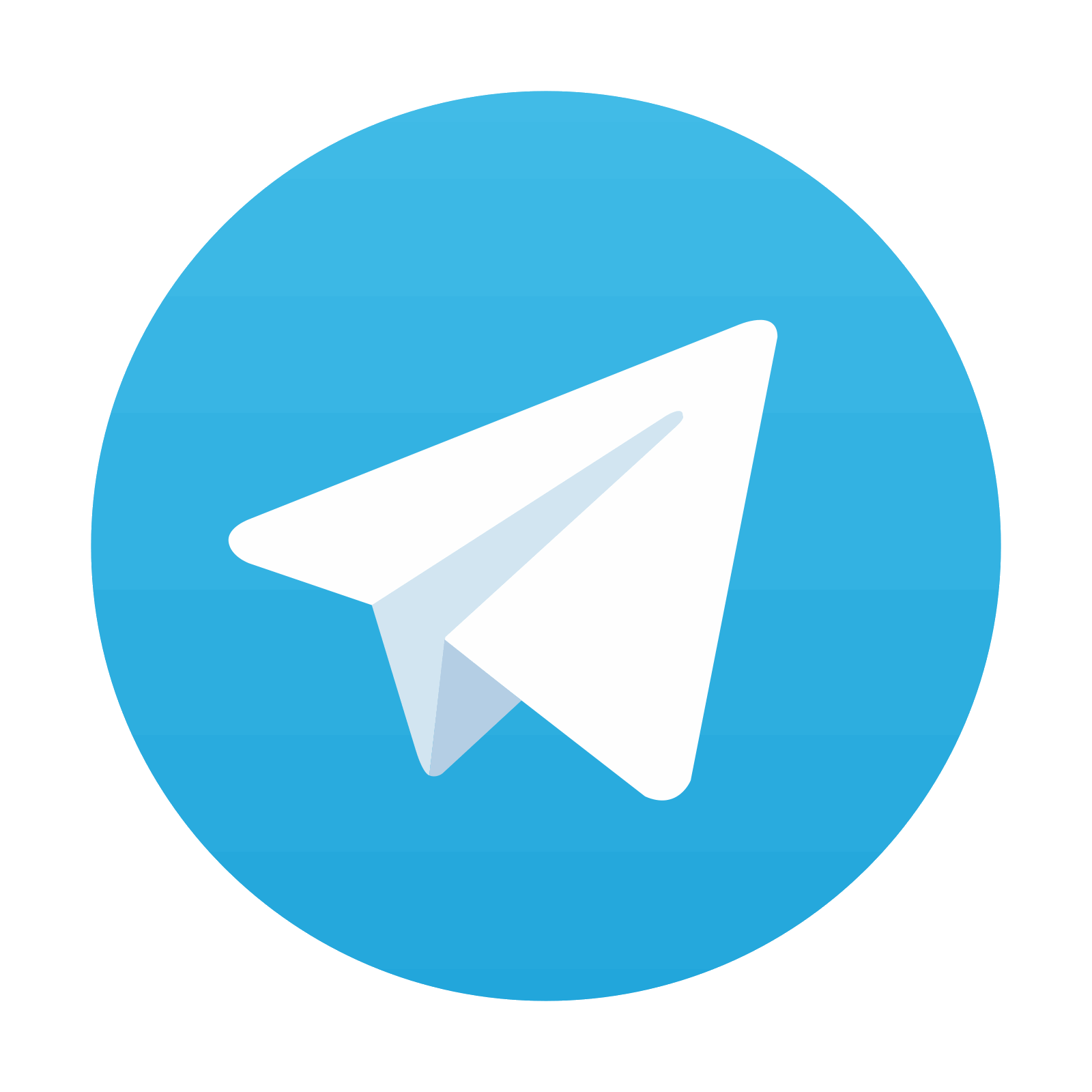
Stay updated, free articles. Join our Telegram channel
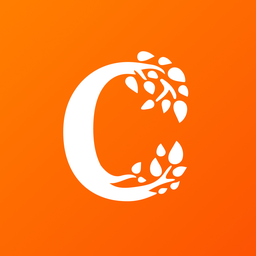
Full access? Get Clinical Tree
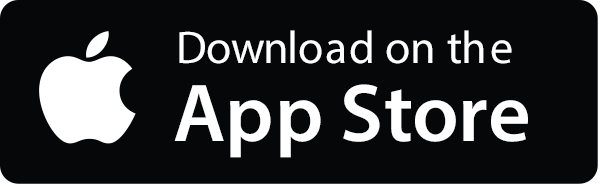
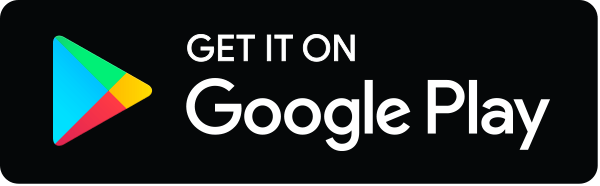