Aminoglycosides and Glycopeptides
Matthijs de Hoog
John N. van den Anker
Aminoglycosides
General Aspects of Aminoglycosides
Aminoglycosides have played a major role in antimicrobial therapy since their discovery in the 1940s (1). Their bactericidal efficacy in gram-negative infections, synergism with β-lactam antibiotics, limited bacterial resistance, and low cost have given these agents a firm place in antimicrobial treatment. However, the successful use of streptomycin (1944), gentamicin (1963), tobramycin (1967), amikacin (1972), and netilmicin (1976) has been complicated by nephrotoxicity and ototoxicity in a significant number of treated patients.
Structure and Chemical Properties
Aminoglycosides comprise two or more amino sugars attached via glycosidic bonds to an aminocyclitol nucleus and have a molecular weight of 445 to 600 daltons. Aminoglycosides can be divided into chemical families with related structures. The relation between the structure of aminoglycosides and their activity is incompletely understood. Aminoglycosides are water-soluble, cationic at normal pH, and are distributed in plasma water. Protein binding is minimal. They have a stable structure over a wide range of temperature and pH (2,3).
Aminoglycosides are inactivated in vitro by concomitant use of β-lactam antibiotics (4,5,6). Tobramycin seems to be more easily inactivated than netilmicin or amikacin. Aminoglycosides act by altering the integrity of the bacterial cell membrane in growing bacteria by way of disturbing protein synthesis through binding to prokaryotic ribosomes (7,8). These cationic antibiotics bind rapidly and passively to the negatively charged parts of phospholipids and other proteins in the bacterial cell membrane and enter the bacterial cell by way of a self-promoted uptake process (9). The uptake process is related to the concentration of aminoglycoside and can be inhibited by low pH, anaerobic conditions, and hyperosmolarity (10,11).
Although inhibition of protein synthesis plays a major part in bacterial cell death, it is not the sole explanation for the bactericidal effect of aminoglycosides. Other antibiotics that inhibit protein synthesis, like chloramphenicol, are only bacteriostatic. Binding of aminoglycosides to the bacterial cell membrane itself may play a role in rapid bacterial cell death (12).
In vitro Activity
Aminoglycosides have a concentration-dependent bactericidal spectrum encompassing aerobic and gram-negative bacteria like Enterobacteriacae, Escherichia coli, Pseudomonas species, and Haemophilus species. The susceptibility of most gram-negative bacteria to gentamicin, tobramycin, netilmicin, and amikacin is relatively similar (13). Although susceptibility to amikacin is three- to fourfold less than that to the other aminoglycosides, this is compensated by its lower toxicity and therefore higher allowable dose. Gentamicin and tobramycin are comparable in activity, although tobramycin is slightly more active against Pseudomonas aeruginosa. They are susceptible to the same modifying enzymes and resistance rates are therefore very similar. In contrast, amikacin is resistant to many of these enzymes, and therefore is often an alternative if strains are resistant to tobramycin or gentamicin. Netilmicin susceptibility is comparable to that of gentamicin and tobramycin, although netilmicin is resistant to some of the gentamicin-inactivating enzymes, and thus in some cases is a good alternative.
The antimicrobial activity of aminoglycosides has four distinct and clinically important aspects: (a) concentration-dependent killing, (b) a postantibiotic effect (PAE), (c) adaptive resistance, and (d) synergism with other antibiotics. In vivo and in vitro studies have shown that the aminoglycoside-induced rate of bacterial killing as well as induction of resistance is peak concentration dependent (14,15,16,17,18). Other in vitro investigations, mimicking in vivo fluctuations of drug concentrations, have shown a single bolus of aminoglycoside to be superior in rate and total amount of bacterial killing to the same dose in a multiple daily-dosing regimen in nonneutropenic animals (19,20).
Aminoglycosides are often reported to have a PAE, meaning that there is suppression of bacterial growth for several hours after antibiotic serum concentrations have dropped below the minimal inhibitory concentration (MIC) (21,22,23). There are discrepancies between in vivo and in vitro studies on this effect, and studies have indicated that PAE is partly determined by diminished regrowth of bacteria at sub-MICs (21). The clinical relevance of the PAE is unclear, and the emphasis on this effect in discussions on extending dose intervals of aminoglycosides is questionable. Synergy of aminoglycosides with other cell-wall-active antibiotics, like penicillins and cephalosporins, has been established (24,25). This synergy is the basis of the clinical choice for combination therapy of aminoglycosides with penicillins or cephalosporins. Issues of toxicity, which will be discussed later, concentration-dependent killing, a PAE (although doubtful), as well as adaptive resistance constitute the rationale for the change to extended-interval dosing of aminoglycosides (26,27,28).
Drug Resistance and Susceptibility
Resistance to aminoglycosides can occur by three mechanisms: ribosomal resistance, decreased uptake and/or accumulation, and enzymatic modification. Ribosomal resistance will not be discussed because it is only pertinent to streptomycin.
Decreased Uptake and/or Accumulation
A decrease in drug uptake is a clinically significant aspect of aminoglycoside resistance. The underlying mechanism, though probably related to membrane impermeability, is not really known (29). It pertains to all aminoglycosides and is a stable characteristic resulting in a moderate level of resistance (30).
Another important phenomenon in aerobic gram-negative bacteria is called adaptive resistance, defined as a reduced antimicrobial killing in originally susceptible bacterial populations after initial incubation with an aminoglycoside (31). It has clinical relevance especially for immunocompromised patients and in serious infections with gram-negative bacteria. Adaptive resistance is probably related to membrane protein changes and altered expression of regulatory genes of the anaerobic respiratory pathway (32). It can be overcome by higher peak serum concentrations of aminoglycosides, which underscores the need for extended dose intervals (33).
Enzymatic Modification
Aminoglycosides can be modified with subsequent loss of antimicrobial activity by enzymes produced by bacterial pathogens (25). The genetic code for these enzymes is largely contained in plasmids, thereby rendering the resistance easily transferable. In addition, it is important to realize that all susceptible positions in aminoglycosides can be modified by several enzymes and that several inactivating genes can easily develop from a common ancestor, implying that it will be unlikely that making aminoglycosides resistant to inactivation by a specific enzyme will be a worthwhile effort (30).
Indications
Aminoglycosides are mainly used for treating serious gram-negative infections caused by enteric bacilli. Aminoglycosides are synergistic with cephalosporins and penicillins in the setting of gram-negative infections and are thus often combined with these cell-wall-active antibiotics (25). They are also used in combination treatment with vancomycin for Staphylococcus aureus (both methicillin-sensitive and methicillin-resistant strains), S. epidermidis, and enterococcal infections (34,35). Oral administration of paromomycin has been successfully used for treating cryptosporidiosis in patients with acquired immune deficiency syndrome (36). In case of empiric treatment with aminoglycosides, local susceptibility patterns have to be taken into account. There are many indications for use of aminoglycosides that are beyond the scope of this chapter. Three conditions will be addressed in more detail: neonatal sepsis, cystic fibrosis (CF), and urinary tract infections.
Neonatal Sepsis
Among major pathogens responsible for bacterial infections during the first month of life, gram-negative bacteria like E. coli, Klebsiella species, Enterobacter species, and Pseudomonas species play an increasing role, possibly related to the increased prenatal administration of antibiotics and use of percutaneous central venous catheters in the neonatal intensive care unit (NICU) (37,38,39,40). Aminoglycosides are effective against most nosocomial-acquired gram-negative infections in term and preterm infants and are synergistic with β-lactam antibiotics in treating group B streptococcal and coagulase-negative staphylococcal infections. They play an important role in the initial empiric treatment of neonatal septicemia (37,38,39,40). Initial treatment combining amoxicillin with gentamicin may be more effective than a combination with cefotaxime (41). After penicillins, aminoglycosides are the most commonly used drugs in the NICU (42). In general, emergence of aminoglycoside-resistant strains other than coagulase-negative streptococci is relatively slow, which is a definite advantage over third-generation cephalosporins (43,44).
Cystic Fibrosis
Acute pulmonary exacerbations of CF are often caused by pseudomonal microorganisms (45). Standard treatment consists of a combination of an aminoglycoside with a β-lactam antibiotic (e.g., ticarcillin) or a quinolone antibiotic and leads to a longer clinical remission than use of a β-lactam alone (46,47). Sequential intravenous/oral ciprofloxacin monotherapy offers a safe and efficacious alternative (48). Higher doses, up to 9 mg per kg per day, are needed because of a higher aminoglycoside clearance in patients with CF (49,50). The safety and efficacy of aerosolized tobramycin maintenance therapy for patients with CF colonized with P. aeruginosa has been demonstrated in several studies (51). Dose advice for inhaled tobramycin is 300 mg twice daily for a period of 28 days (52). Systemic bioavailability is approximately 12% and monitoring serum concentrations in patients with renal failure is indicated (53). Once-daily and three times daily dosing in pediatric patients with CF is equally effective and less nephrotoxic (54).
Urinary Tract Infections
Aminoglycosides are excreted by way of glomerular filtration and are partly actively reabsorbed, leading to high parenchymal as well as urine concentrations. This makes these drugs useful in treating acute pyelonephritis and cystitis. Even single doses lead to prolonged periods of adequate concentrations in urine (55,56). Once-daily dosing (ODD) of gentamicin is effective in treating urinary tract infections in children (57).
Pharmacokinetics
Aminoglycosides have a pharmacokinetic profile consisting of a rapid distribution phase (t1/2α), an elimination phase (t1/2β), and a second elimination phase (t1/2γ). The gamma phase can only be determined after discontinuation of the drug. Distribution half-life is 5 to 10 minutes in adults but has never been measured in newborns. The gamma phase in infants is long. Netilmicin was detectable in blood and urine 11 and 14 days after discontinuation, with a t1/2γ of 62.4 hours (58). The tissue half-life in renal cortex is 4 to 5 days (59). In general, the serum concentrations and pharmacokinetic data determined in most studies are derived from the elimination phase, which is adequately described by a one-compartment model. There are, however, some studies that have shown a two-compartment model to be superior in predicting serum t1/2β and serum concentrations (60,61,62,63).
Because aminoglycosides are distributed over extracellular water, there are age-related changes in volume of distribution (Vd) dependent on the decrease of total body water with age. The largest Vd is encountered in premature neonates. Tables 30.1 and 30.2 display age-related pharmacokinetic parameters for aminoglycosides in neonates, infants, and children.
Pharmacokinetics in Neonates
Many studies in neonates have been performed, and therefore only larger studies in this age group are included. In larger studies Vd of gentamicin ranges from 0.70 L per kg in neonates with a gestational age (GA) less than 32 weeks to 0.32 L per kg in children aged 11 to 18 years (64,65,66).
The Vd of most drugs is larger in neonates, especially in prematures, primarily due to a higher percentage of extracellular water (67,68). As can be seen in Table 30.1, this also holds true for aminoglycosides. There is a consistently higher Vd for prematures, especially in the group with very low birth weight (VLBW)/GA less than 30 weeks. Most authors have found birth weight (BW) to be the best predictor of Vd (62,69,70,71,72), although some have found Vd to be independent of GA (66,73). In practice, this means that prematures will end up having lower peak serum concentrations. The interpatient variability of Vd in these groups is greater, and therefore serum concentrations are difficult to predict in the individual premature infant. Total body clearance (CL), associated with GA (62,69,74) and BW (62,69,70,72), is lower, and t1/2β is longer, in preterm infants, leading to higher serum trough concentrations in this group. This can be explained by the significant increase of glomerular filtration rate (GFR) with GA and BW described in recent studies (75,76). CL and t1/2 are also highly variable in VLBW infants. Substantial effort has been put into developing equations, mostly based on population pharmacokinetic studies, which potentially will lead to better prediction of serum concentrations in individual patients (70,72,73). In practice, these equations are not able to adequately predict variability and subsequent serum concentrations in the same patient (64).
Diminishing extracellular fluid in the neonatal period (77) leads to a corresponding decrease in Vd with increasing postnatal age (PNA), again especially in the VLBW group. Recent data have shown a significant postnatal increase in GFR (75,76). A concomitant change in CL and t1/2 has been shown for amikacin (78), gentamicin (73,74,79,80,81), netilmicin (58,62), and tobramycin (82), but this result has been refuted by others (71,83).
Patent ductus arteriosus (PDA) as well as postnatal exposure to indomethacin or other nonsteroidal anti-inflammatory drugs alters the rapid postnatal increase in GFR (84,85), possibly through decreased renal blood flow, leading to an increase in Vd and a reduction of CL. Increase in Vd of gentamicin is found in infants with PDA (66,86). In this patient group fluid overload is common. Closure of PDA leads to significant decrease in Vd of more than 30% (86,87). Dosage adjustments, based on therapeutic drug monitoring (TDM), should be made in patients with PDA. The effect of indomethacin, used for closure of PDA, as well as surgical closure itself necessitates TDM. Information on gentamicin disposition in infants on extracorporeal membrane oxygenation (ECMO) is scarce. Two small studies (63,88) showed Vd to be increased. Serum half-life in patients on ECMO was 9.55 and 9.24 hours, respectively, and decreased to 3.87 hours in the same patients off ECMO in the study by Dodge et al. (88). On the basis of these data, dosage adjustments should be made in this group of infants. Prenatal exposure to corticosteroids, which is seen increasingly in the VLBW group, leads to increased intrauterine maturation of kidney function, possibly through direct vasodilation mediated by glucocorticoid receptors (76,89). Although this point has not been investigated, it might have a significant effect on pharmacokinetic parameters in this group of infants. These studies indicate that extra TDM is warranted in patients who are on ECMO, have an open ductus Botalli, or are exposed to indomethacin. Aminoglycosides are eliminated from the body by way of glomerular filtration; it is therefore predictable that a relation between GFR and serum concentrations exists. The link between GFR and aminoglycoside pharmacokinetics is often (58,71,73,74,80,90,91,92), but not consistently (62,80,93,94), described in neonates. Furthermore, in adults it has been shown that aminoglycoside concentrations can change without concomitant change of serum creatinine (95). Keyes et al. (93) showed that serum trough concentrations could not be reliably predicted in newborns with serum creatinine. In conclusion, these studies suggest that, though there is a relation, serum creatinine in the first week of life cannot be accurately used to predict aminoglycoside CL.
Pharmacokinetics in Infants and Children
There are also age-related differences in aminoglycoside pharmacokinetics in infants and older children. Figure 30.1 shows that Vd (for amikacin) decreases with increasing PNA and CL decreases with increasing PNA (96). Changes in both Vd and CL are most pronounced in the first 2 weeks of life. Vd decreases by one-third when comparing patients younger and older than 3 months (97). A concomitant change in peak serum concentrations with age is seen (98). It is questionable whether these changes are clinically relevant for dose or dose interval in pediatric patients beyond the neonatal age.
Table 30.1 Results of Pharmacokinetic Studies of Aminoglycosides in Neonates | ||||||||||||||||||||||||||||||||||||||||||||||||||||||||||||||||||||||||||||||||||||||||||||||||||||||||||||||||||||||||||||||||||||||||||||||||||||||||||||||||||||||||||||||||||||||||||||||||||||||||||||||||||||||||||||||||||||||||||||||||||||||||||||||||||||||||||||||||||||||||||||||||||||||||||||||||||||||||||||||||||||||||||||||||||||||||||||||||||||||||||||||||||||||||||||||||||||||||||||||||||||||||||||||||||||||||||||||||||||||||||||||||||||||||||||||||||||||||||||||||||||||||||||||||||||||||||||||||||||||||||||||||
---|---|---|---|---|---|---|---|---|---|---|---|---|---|---|---|---|---|---|---|---|---|---|---|---|---|---|---|---|---|---|---|---|---|---|---|---|---|---|---|---|---|---|---|---|---|---|---|---|---|---|---|---|---|---|---|---|---|---|---|---|---|---|---|---|---|---|---|---|---|---|---|---|---|---|---|---|---|---|---|---|---|---|---|---|---|---|---|---|---|---|---|---|---|---|---|---|---|---|---|---|---|---|---|---|---|---|---|---|---|---|---|---|---|---|---|---|---|---|---|---|---|---|---|---|---|---|---|---|---|---|---|---|---|---|---|---|---|---|---|---|---|---|---|---|---|---|---|---|---|---|---|---|---|---|---|---|---|---|---|---|---|---|---|---|---|---|---|---|---|---|---|---|---|---|---|---|---|---|---|---|---|---|---|---|---|---|---|---|---|---|---|---|---|---|---|---|---|---|---|---|---|---|---|---|---|---|---|---|---|---|---|---|---|---|---|---|---|---|---|---|---|---|---|---|---|---|---|---|---|---|---|---|---|---|---|---|---|---|---|---|---|---|---|---|---|---|---|---|---|---|---|---|---|---|---|---|---|---|---|---|---|---|---|---|---|---|---|---|---|---|---|---|---|---|---|---|---|---|---|---|---|---|---|---|---|---|---|---|---|---|---|---|---|---|---|---|---|---|---|---|---|---|---|---|---|---|---|---|---|---|---|---|---|---|---|---|---|---|---|---|---|---|---|---|---|---|---|---|---|---|---|---|---|---|---|---|---|---|---|---|---|---|---|---|---|---|---|---|---|---|---|---|---|---|---|---|---|---|---|---|---|---|---|---|---|---|---|---|---|---|---|---|---|---|---|---|---|---|---|---|---|---|---|---|---|---|---|---|---|---|---|---|---|---|---|---|---|---|---|---|---|---|---|---|---|---|---|---|---|---|---|---|---|---|---|---|---|---|---|---|---|---|---|---|---|---|---|---|---|---|---|---|---|---|---|---|---|---|---|---|---|---|---|---|---|---|---|---|---|---|---|---|---|---|---|---|---|---|---|---|---|---|---|---|---|---|---|---|---|---|---|---|---|---|---|---|---|---|---|---|---|---|---|---|---|---|---|---|---|---|---|---|---|---|---|---|---|---|---|---|---|---|---|---|---|---|---|---|---|---|---|---|---|---|---|---|---|---|---|---|---|---|---|---|---|---|---|---|
|
Table 30.2 Results of Pharmacokinetic Studies of Aminoglycosides in Infants and Children | ||||||||||||||||||||||||||||||||||||||||||||||||||||||||||||||||||||||||||||||||||||||||||||||||||||||||||||||||||||||||||||||
---|---|---|---|---|---|---|---|---|---|---|---|---|---|---|---|---|---|---|---|---|---|---|---|---|---|---|---|---|---|---|---|---|---|---|---|---|---|---|---|---|---|---|---|---|---|---|---|---|---|---|---|---|---|---|---|---|---|---|---|---|---|---|---|---|---|---|---|---|---|---|---|---|---|---|---|---|---|---|---|---|---|---|---|---|---|---|---|---|---|---|---|---|---|---|---|---|---|---|---|---|---|---|---|---|---|---|---|---|---|---|---|---|---|---|---|---|---|---|---|---|---|---|---|---|---|---|
|
Vd and total plasma CL for aminoglycosides are increased in patients with CF, necessitating larger doses in this patient group (46,49,50).
A relation between GFR and aminoglycoside CL in pediatric patients has been established, and aminoglycoside dosing has to be adjusted according to creatinine CL (99,100).
Efficacy
Efficacy of aminoglycosides is related to both the peak serum concentration-to-MIC ratio (peak/MIC) and the area under the time-versus-concentration curve (AUC/MIC) in clinical and experimental studies (14,17,101). Peak/MIC ratios of 5 to 10 are desirable for clinical efficacy. In the neonatal period aminoglycosides are administered in combination treatment of suspected neonatal sepsis. Monitoring the efficacy of antibiotic treatment in neonates is difficult. Culture-proven early-onset sepsis occurs in approximately 2% of VLBW infants, but there are limitations to blood cultures in neonates, and single blood cultures can be false negative (102,103). Furthermore, increasing prenatal treatment of mothers with antibiotics obscures culture results in newborns. Early detection of neonatal sepsis remains difficult. Laboratory tests are unspecific and clinical signs can be ambiguous. Neonatal sepsis is suspected in many VLBW infants on clinical grounds, and antibiotic treatment is frequently started and discontinued after 48 to 72 hours when blood cultures and results of other tests remain negative (104). Given the infrequent occurrence of positive blood cultures, these cannot be used as a marker for antibiotic efficacy. Laboratory data, including C-reactive protein, complete blood cell count, and ratio of immature to total neutrophils, lack sufficient sensitivity to detect neonatal sepsis and can therefore not be used to evaluate efficacy (105). The same counts for clinical features (e.g., respiratory rate, skin color), which either cannot be quantified or lack a clearly defined relation between predictor and outcome.
In recent years aminoglycoside dosing in children has changed to ODD. Although theoretically this should lead to an increase of efficacy and a decrease of toxicity, this has not been clearly demonstrated in adults and has not been extensively studied in infants and children. Most studies did not address dose–response effect; some studies compared ODD to multiple daily doses (MDD) (106,107). In these studies both efficacy and toxicity were comparable. Urinary tract infections were effectively treated with ODD or thrice-daily dosing (TDD) of gentamicin in 179 pediatric patients (106). Severe bacterial infections in 52 pediatric patients were equally effectively treated with ODD and TDD of gentamicin in combination with a β-lactam antibiotic. Fever as well as C-reactive protein decreased comparably in both groups (107). ODD treatment of febrile children undergoing stem cell transplantation showed a tendency for less nephrotoxicity and more efficacy (108). The combination of amikacin with a β-lactam antibiotic showed satisfactory clinical results in 98% of severe bacterial infections in 56 infants and children (109).
Toxicity
The major specific side effects of aminoglycosides are nephrotoxicity and ototoxicity. Neurotoxicity by way of blockade of neuromuscular synapses with prolonged muscle weakness after the use of muscle relaxants has not been described in infants. The delayed-type hypersensitivity reaction of the skin is mostly seen after use of topical application of neomycin or framycetin and has not been described in neonates. Nephro- and ototoxicity will be described in further detail.
Nephrotoxicity
Aminoglycoside nephrotoxicity is induced by way of proximal tubular injury leading to cell necrosis. The mechanisms of toxicity have been mostly studied in animals, where it was shown to induce glomerular and tubular damage both pre- and postnatally (110). Less than 5% of filtered aminoglycosides binds to the brush-border membrane of proximal tubular cells and is actively reabsorbed, finally causing cell death. The degree of nephrotoxicity is determined by the quantity of aminoglycosides stored in the proximal tubular cell and the intrinsic potency of the drug to damage subcellular structures (111). In several large meta-analytical studies toxicity seems to be related to high trough concentrations, indicating that these concentrations are not low long enough to prevent renal accumulation. Nephrotoxicity related to ODD as compared with MDD was found to be equal or less in these studies (26,27,112,113,114). A recent prospective study in adults showed that both probability and time of occurrence of nephrotoxicity are negatively influenced by MDD (115). There is no clear distinction in level of nephrotoxicity among the four aminoglycosides in studies in adults (59).
The incidence of aminoglycoside nephrotoxicity in neonates is not well known but seems to be considerably lower than in adults. Enduring glomerular filtration impairment has not been conclusively shown in prospective studies (116,117,118,119). No difference in renal function was found between ODD and MDD for amikacin and gentamicin (117,120).
Reversible tubular dysfunction has been shown in many studies involving neonates (116,118,119,121,122,123,124,125) and is more pronounced in term infants than in preterm newborns (117,118,124), which is possibly explained by maturity-dependent blood supply differences of the outer parts of the kidney and lower binding constants in the immature kidney (126). In infants with a GA greater than 34 weeks no difference in proximal tubular damage was found between ODD and MDD of amikacin (117). Urinary electrolyte loss is higher at peak serum concentrations (116,121). In the ill term and especially preterm infant, who is already at risk for electrolyte disturbances, the increased loss during aminoglycoside therapy might be clinically relevant and warrants extra monitoring (127).
Ototoxicity
Aminoglycosides are potentially cochleo- and vestibulotoxic. They accumulate in the lymphatic fluid of the inner ear, from which they are only slowly eliminated (24 to 36 hours) (131). There is evidence for saturable uptake in animals (132). Certain gene mutations lead to a familial increased risk of aminoglycoside-induced sensorineural hearing loss (133). Sequentially outer hair cells, inner hair cells, and spiral ganglional neurons are damaged. Aminoglycosides seem to give a polyamine-like enhancement of glutamate N-methyl-D-aspartate receptor activity, resulting in excessive entry of sodium and calcium, which leads to excitotoxic cell death (134). Hearing loss is usually bilateral, symmetrical, and permanent, and can also have a delayed onset of months (131). Most authors suggest that ototoxicity is related to total dose and duration of therapy rather than to serum aminoglycoside serum concentrations, but the relation to aminoglycoside serum concentrations remains unclear. This form of toxicity usually occurs in patients who have received either long or repeated courses of aminoglycosides (135). No difference in incidence between ODD and MDD could be demonstrated (112,115). In experimental studies, amikacin appears to be more cochleotoxic than do gentamicin and tobramycin. Netilmicin is probably the least ototoxic aminoglycoside (129). Although vestibulotoxicity is a disabling side effect in adults, it has not been shown in neonates.
There are many pitfalls in relating use of aminoglycosides to loss of hearing in infants. Hearing loss in neonates occurs in 0.1% to 0.3% of cases (136). Numerous risk factors for neonatal hearing loss have been identified. Perinatal infections, meningitis, prematurity, hyperbilirubinemia, BW less than 1,500 g, asphyxia, respiratory distress syndrome, mechanical ventilation, antibiotics, and diuretics have all been incriminated (137). Even though some studies show a relation to administration of aminoglycosides, it remains difficult to separate the effect of aminoglycoside use from concomitant factors (136).
Ototoxicity is an infrequent occurrence in neonatal studies. Many studies did not find any aminoglycoside-related toxicity. In some studies delayed onset of hearing loss has been described (138,139). Several studies found a transient hearing loss (140,141). Some studies found a relation with duration (137,142) and total dose (137,143). Recently, the relation between exposure to tobramycin and the risk of detecting hearing loss with hearing screening was studied (144). Exposure to tobramycin in terms of treatment duration, total dose, or serum concentrations was not related to a failure to pass hearing screening. Cotreatment with vancomycin or loop diuretics was not associated with an increased chance of hearing loss in this study as in other studies (117,129,144,145). The results lead us to conclude that aminoglycoside-related hearing loss in infants is infrequent, possibly transient, and might be late in appearing. No clear relation was found to peak and trough concentrations. Ototoxicity in infants and children is infrequent as well, although it was not often explicitly studied. Recent studies investigating ODD and MDD of aminoglycosides did not detect any ototoxicity (107,109,130). Other studies only found transient hearing loss (146,147). In contrast, hearing loss was demonstrated in pediatric patients with CF exposed to prolonged and/or repeated aminoglycoside treatment, also with inhalation therapy (53,148,149).
Aminoglycoside Therapeutic Drug Monitoring and Dosing
Dose and dosing interval are determined by the desired therapeutic range and pharmacokinetic as well as pharmacodynamic properties of a drug. It is difficult to define the desired therapeutic range for aminoglycosides. Peak concentrations of greater than 4 to 5 mg per L are generally accepted as necessary for antibacterial efficacy when dosing thrice daily (17,95,150,151). Efficacy of aminoglycosides is related to the ratio of peak serum concentration to the MIC of the infecting microorganism and the AUC. In vitro ratios of 10:1 prevent emergence of aminoglycoside-resistant pathogens (14,17). Commonly accepted trough concentration goals in adults are less than 2 mg per L, but when dosing once a day, most authors keep less than 1 mg per L as a safe limit (28,152,153). Based on the aminoglycoside susceptibility of gram-negative pathogens involved in neonatal septicemia, a reasonable target range for neonates would therefore be peak serum concentrations of 5 to 10 mg per L for gentamicin, netilmicin, and tobramycin and 15 to 30 mg per L for amikacin. Trough concentration goals are less than 2 mg per L when dosing thrice daily and less than 0.5 to 1.0 mg per L for ODD in gentamicin, netilmicin, and tobramycin and 1.5 to 3 mg per L for amikacin.
Neonates
Aminoglycoside dosing in newborns has been revised toward larger doses at extended intervals (1,12). A substantial reduction in inter- and intraindividual variation of serum concentrations can be achieved with the use of dedicated pediatric vials as has been demonstrated for amikacin (154). Recent studies have demonstrated that serum concentrations as described in the foregoing can be reached with doses of 3.5 to 5 mg per kg (gentamicin, tobramycin, netilmicin) and 7.5 to 20 mg per kg (amikacin) (81,85,120,155,156,157,158,159,160,161). From the viewpoint of efficacy it is important to obtain an adequate peak serum concentration after the first dose, which has led several authors to advise a loading dose of aminoglycosides (87,153,160,162). This goal can also be reached without loading doses (64,155). Dosing intervals range from 12 to 48 hours, depending on GA and PNA.
Routine TDM for aminoglycosides is normally performed around the third or fourth dose and is based on the assumption that steady state is more or less attained. For several reasons this assumption is not valid in neonates in the first week of life. Antibiotic courses in neonates are often discontinued after a few days when blood cultures and other tests remain negative. Because extended-interval (24 to 48 hours, depending on GA) dosing of aminoglycosides is now a general practice, steady state would not be reached before discontinuation of the drug, and TDM would therefore not be performed in time to be of use. Using population pharmacokinetic principles and Bayesian feedback it was postulated that two serum samples after the first dose (1 hour and 12 to 18 hours) could help optimize TDM, but this still needs to be validated (165). Efficacy and toxicity have not been clearly related to peak or trough serum concentrations with extended dose intervals, and clinically important nephro- and ototoxicity are rare in neonates in courses shorter than 7 days. Furthermore, subsequent serum concentrations cannot be adequately predicted in this age group (15,64). The importance of routine TDM in the first week of life for efficacy and toxicity reasons has therefore been questioned. An exception should be made for neonatal patients with renal failure, patients with obvious neonatal asphyxia (e.g., 5′ Apgar score of <5 at 5 minutes), and patients exposed to drugs or situations that are known to significantly alter pharmacokinetic behavior (e.g., indomethacin, ECMO). In these difficult-to-manage patients use of a population model with feedback of repeated serum concentrations as well as repeated serum creatinine might be useful in guiding therapy.
Infants and Children
Aminoglycoside dosing in infants and children has changed toward ODD over the last decade. A number of studies have evaluated ODD versus TDD, with daily doses varying from 4.5 to 7.5 mg per kg (106,130,146,166). These studies contain a heterogeneous patient population of serious gram-negative infections and urinary tract infections. Mean peak concentrations obtained with ODD were 9.8 to 20.4 mg per L and sufficient for most microorganisms to obtain a peak/MIC ratio of greater than 10. Mean trough concentrations range from 0.18 to 0.5 mg per L. A meta-analysis has shown similar results in efficacy and toxicity for ODD versus TDD (167).
Routine TDM is normally performed around the third or fourth dose. Predicting future serum concentrations in a pediatric population with the help of a Bayesian feedback model is possible (168,169). The necessity of routine TDM in pediatric patients with normal renal function and receiving short courses (<10 days) is still questionable (170,171).
Glycopeptides
General Aspects of Glycopeptide Antibiotics
Glycopeptide antibiotics are a group of antibiotics with related structure and similar antimicrobial activity. The two major clinically important compounds in this group are vancomycin and teicoplanin. Vancomycin is an antibiotic first isolated from an Indonesian jungle soil sample in 1956, and was the first of the class of glycopeptide antibiotics. It was largely supplanted by methicillin sodium, introduced in 1960, because of the frequent occurrence of side effects associated with vancomycin use, including generalized skin eruptions, phlebitis, fever, and, more importantly, deafness and renal failure (172,173). Reduction of impurities and a concomitant decrease in incidence of side effects as well as the emergence of methicillin-resistant staphylococci have led to a resurgence of vancomycin use (174,175,176). Teicoplanin was introduced in 1982.
Despite the emergence of vancomycin-resistant enterococci, vancomycin still is the most widely used glycopeptide antibiotic and is a cornerstone in antibiotic treatment of gram-positive infections in adults, children, and neonates.
Structure and Chemical Properties
Vancomycin and teicoplanin are the commonly used glycopeptide antibiotics and are unrelated to other antibiotics. They are complex soluble glycopeptides, consisting of a seven-membered peptide chain, in the form of three large rings. Five of the seven amino acid residues are common to all glycopeptides (177,178,179). A disaccharide, composed of glucose and vancosamine, is also present but is not part of the cyclic structure. The molecular weight of vancomycin is 1,448 daltons (180).
Vancomycin is hydrophobic but less so than teicoplanin (179). It has a moderate protein binding in adults (10% to 55%) and exerts its activity over a wide pH range of 6.5 to 8 (181,182). Protein binding in neonates is higher (72% to 81%), but binding to bilirubin is not concentration dependent indicating a low possibility of an increase in free bilirubin (183). Vancomycin can be inactivated by heparin in high concentrations (184). Teicoplanin can be added to parenteral solutions (185).
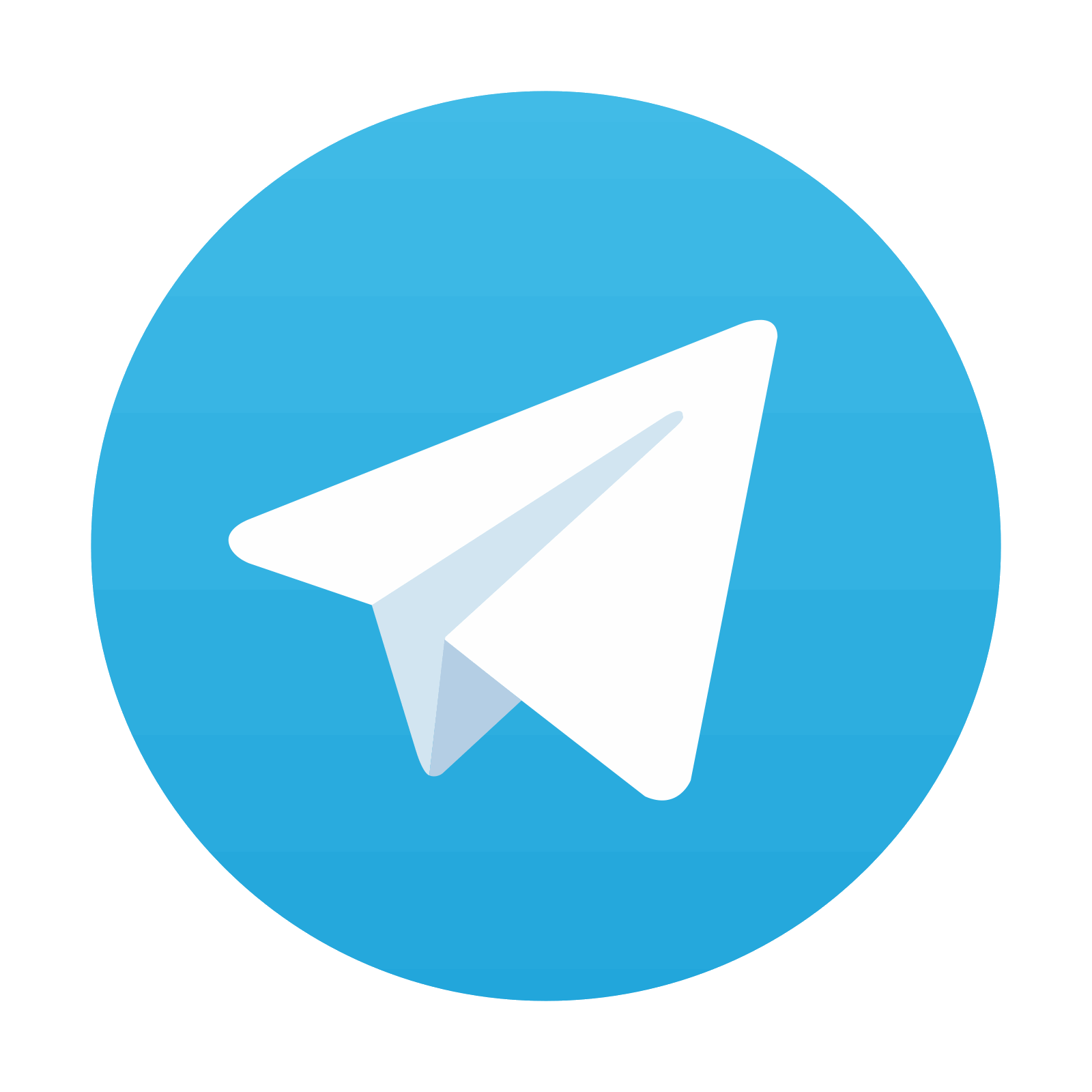
Stay updated, free articles. Join our Telegram channel
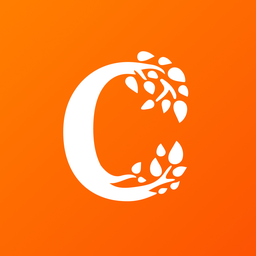
Full access? Get Clinical Tree
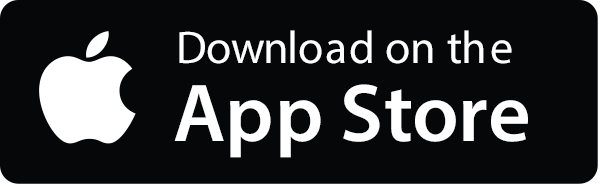
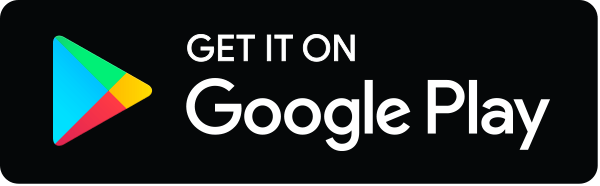