Abstract
The placenta develops alongside the embryo and fetus and is responsible for fetal gas exchange and nutrition. The placenta also has important immune and endocrine functions and thus undertakes to fulfill the roles played by various somatic organs in the post-natal situation (Figure 5.1). The placental membrane, the chorion, prevents the fetal and maternal blood from mixing, while allowing transport of molecules. The human placenta is haemochorial, which means that maternal blood contacts the chorionic placental membrane (fetal epitheliem).
Introduction
The placenta develops alongside the embryo and fetus and is responsible for fetal gas exchange and nutrition. The placenta also has important immune and endocrine functions and thus undertakes to fulfill the roles played by various somatic organs in the post-natal situation (Figure 5.1). The placental membrane, the chorion, prevents the fetal and maternal blood from mixing, while allowing transport of molecules. The human placenta is haemochorial, which means that maternal blood contacts the chorionic placental membrane (fetal epitheliem). Molecules traverse the placental barrier either via passive diffusion down their concentration gradient or by active transport.
Figure 5.1 The placenta is the organ of respiratory gas exchange for the fetus. It supports the developing fetus by transferring oxygen and nutrients from the maternal to the fetal circulation and transferring carbon dioxide and wastes away from the fetal to the maternal circulation. In addition to gas exchange, the placenta also performs endocrine functions, supporting the pregnancy by the production of hormones.1 Adapted from reference 2.
Fully oxygenated and nutrient-rich blood is delivered to the placenta by the maternal circulation via the uterine arteries that supply the numerous arterioles (spiral arteries) in the endometrium, which open into the intervillous space. Deoxygenated blood and waste products from the fetus are carried out of the maternal placental circulation through the endometrial and then into the uterine veins. The intervillous space is where the chorionic villi housing the umbilical capillaries float. The maternal blood in the intervillous space is a mixture of incoming fully oxygenated and deoxygenated blood due to placental oxygen uptake, resulting in an intervillous pool of blood with oxygen partial pressure (PO2) that is relatively lower than that of maternal arterial blood. Essentially, the mixed maternal blood in the intervillous space forms the supply of oxygen to the fetus. Gas exchange takes place between maternal and fetal blood through the chorion. Nutrient-rich and relatively well-oxygenated blood in the fetal capillaries of the placenta is delivered to the fetus via the umbilical vein and deoxygenated, lower nutrient blood returns back to the placenta from the fetus via the umbilical arteries. Thus, placental oxygen transfer to the fetus is a three-step process: (1) maternal uterine arterial supply, (2) transfer of oxygen across the placental membrane and (3) fetal oxygen uptake in the umbilical vein (Figure 5.2).
Figure 5.2 A simplified diagram of the placental circulation. Fully oxygenated maternal blood enters the intervillous space in the placenta via the numerous uterine arterioles supplied by the uterine arteries and maternal deoxygenated blood exits the placenta via the uterine venuoles, which anastomose with the uterine veins. Exchange of gases and other molecules occurs through the placental chorionic membrane that forms the villi bathed in maternal blood. Relatively well-oxygenated blood in the fetal capillaries is delivered to the fetus via the umbilical vein and deoxygenated fetal blood returns to the placenta through the chorionic/basal plate via the umbilical arteries.
This chapter will begin by explaining the factors that affect placental oxygen transfer across the placenta. Maternal and fetal placental blood flow will be discussed in normal, growth-restricted and high-altitude pregnancies. Then, the role of magnetic resonance imaging (MRI) as a new and novel method of quantifying placental gas exchange will be discussed. Lastly, the circulatory changes at birth from the change in gas exchange from the placenta to the neonatal lungs will be briefly described.
The data presented in this chapter on placental oxygen transport is based primarily on animal studies, with most data from chronically catheterized sheep and some from other species such as monkeys, rabbits and guinea pigs. Measurements in sheep and other laboratory animals are obtained from blood samples drawn from surgically implanted catheters in umbilical and uterine vessels. Blood flow measurements are obtained using various techniques such as fluorescent3,4 or radionuclide5,6-labelled microspheres and flow probes7–9 in these surgically prepared animals. The limited human blood gas data is from blood samples obtained during caesarean section, at delivery or from cordocentesis, whereby a needle is inserted through the pregnant abdomen and into the umbilical vessels under the guidance of ultrasound imaging.10 The blood flow data in humans is obtained primarily with Doppler ultrasound11,12 and recently with MRI.13–15
Factors Affecting Placental Gas Exchange Across the Placenta
Transfer of oxygen across the placenta is influenced by characteristics of maternal and fetal blood, such as blood flow and oxygen content in both the maternal and the fetal circulation, and diffusion characteristics of the placental membranes. The main factors that influence placental oxygen transfer are: (1) placental membrane diffusing capacity, (2) maternal and fetal arterial PO2, (3) maternal and fetal blood haemoglobin oxygen affinity and (4) maternal and fetal blood flow rates.16 Other factors that can influence gas exchange are vascular development and spatial arrangement (e.g. vascular shunts) in the fetal and maternal compartments of the placenta, the direction of maternal and fetal blood flows, and features of placental membranes affecting diffusion capacity.17
The placental microvasculature is complex and measuring gas exchange at the level of the functional subunits (or exchange units) is nearly impossible in vivo. Diffusing capacity and/or fetal and maternal blood flow rates along the length of the placental ex-change membrane are non-uniformly distributed.16,17 Thus, blood in different compartments of the placenta can have varying PO2 and partial pressure of carbon dioxide (PCO2). Coupled with the presence of vascular shunts, it is technically challenging to measure and interpret the uterine and umbilical venous outputs within the placenta that contain mixed blood from various regions of the placenta with varying gas exchange rates in comparison to maternal and fetal arterial inputs. Figure 5.3 presents the mean normal values of blood gases, pH and haemoglobin in the blood of the main fetal and maternal vascular inputs and outputs of the placenta. Few studies have quantified blood gases simultaneously in both the main fetal and maternal inputs and outputs of the placenta. Therefore, Figure 5.3 combines data from studies investigating either the maternal or fetal sides of the placenta in late gestation.
Figure 5.3 Normal human maternal uterine artery16,18,19 and vein16,20–24 and fetal umbilical16,20,22–32 artery and vein blood gases, haemoglobin and pH in late gestation. PO2 = partial pressure of oxygen; SO2 = oxygen saturation; PCO2 = partial pressure of carbon dioxide; Hb = haemoglobin; O2 capacity = 1.36 × Hb; O2 content = 1.36 × Hb × SO2, where 1.36 is the amount of O2 in ml bound to a gram of haemoglobin at 1 atm. The values are averaged from the literature data. The data presented comes from studies that collected maternal venous and fetal umbilical arterial and venous blood samples by cordocentesis, during caesarean section or after normal delivery.
Placental Diffusing Capacity
Oxygen and carbon dioxide are small, neutrally charged particles and traverse the placental membrane (polarized lipid bilayer) via simple passive diffusion down their concentration gradient. In contrast, large and charged particles like glucose transfer across the placenta by facilitated diffusion (i.e. via binding with carrier proteins), or amino acids by active transport. The diffusion capacity varies along the length of the placental membrane and is affected by both thicknesses and permeability. The amount of oxygen flowing across the placental membrane can be approximately modelled using Fick’s first law16,17:

where dQdt is the amount of substance Q, such as oxygen, traversing the placental membrane per unit time t, A is the area of exchange, D is diffusion constant (cm2/s), ΔC is the volumetric concentration difference across the membrane and ΔX is the diffusion distance (the membrane thickness). Thus, the flow of oxygen is dependent on diffusion characteristics and partial pressure gradient across the membrane.
Since thickness and permeability vary along the length of the placental membrane, to calculate the membrane diffusivity at a particular location, the terms on the right side of Equation (5.1) can be assumed constants and joined into a single term, Dp, to represent the placenta membrane diffusing capacity in ml/min/partial pressure difference for a given gas16,17:

where V· is the amount of gas transferred across the placental membrane per unit time and p¯m−p¯f is the mean difference in partial pressure of the gas in maternal, m, and fetal, f, blood. In Equation (5.2), partial pressure difference is more applicable for respiratory gases instead of concentration difference stated in Fick’s first law in Equation (5.1).
Oxygen cannot be used to compute its placental diffusing capacity (DpO2) due to two factors: (1) a significant amount of oxygen is actively consumed by the placental tissue and (2) fetal oxygen uptake is limited by blood flow and not diffusion, and there are inequalities in maternal and fetal blood flows along the length of the placental membrane.16,17 This results in an underestimation in the calculation of the placental oxygen diffusion capacity as the blood sampled on both sides of the placenta consists of mixed venous outputs. To overcome this, researchers have used a metabolically inert gas, carbon monoxide, as a proxy of placental oxygen diffusing capacity in animal models. Carbon monoxide binds with haemoglobin, but is not consumed by the placenta and thus its exchange is limited by diffusion.33,34 The placental diffusing capacity of carbon monoxide (DpCO) can be calculated as follows:

where V˙CO is the amount of CO transferred across the placenta to the fetus per unit time (fetal CO uptake) and P¯mCO−P¯fCO
is the difference in mean partial pressures of carbon monoxide between maternal and fetal blood across the placenta. Table 5.1 shows measured DpCO in various species in late gestation. Placental diffusing capacity increases with gestational age as evidenced by measured increase in DpCO by ~350%, from 0.0413 to 0.1858 ml/min/mmHg between 45–50 to 58–68 days’ gestation in guinea pigs (term 70 days).35 This significant increase accommodates the increased fetal demand for oxygen resulting from increasing fetal weight and is associated with an increase in total placental vascular volume and decreasing mean diffusion distance.36 A parallel increase in DpCO and fetal weight over the course of gestation implies that placental diffusing capacity does not change significantly when indexed to fetal weight.37 Based on DpCO measurements and comparing the relative reaction and diffusion rates of oxygen and carbon monoxide, the DpO2 has been estimated to be about 1.2 to 2 times higher than DpCO, in the range of 0.7 to 1.1 ml/min/mmHg/kg33. There is no reliable DpCO data available in humans38 because this data can only be collected using invasive methods at this time.
Table 5.1 Interspecies differences in placental carbon monoxide diffusing capacity near-term(mean ± SEM)
DpCO (ml/min/mmHg/kg fetal weight) | DpCO (ml/min/mmHg/kg placental weight) | Number of cell layers separating maternal and fetal blood17 | |
---|---|---|---|
Sheep39 | 0.55 ± 0.02 | 3.0 ± 0.4 | 5 |
Monkey37 | 0.65 ± 0.06 | 1.6 ± 0.2 | 3 |
Dog33 | 0.57 ± 0.80 | − | 4 |
Guinea Pig35 | 2.28 ± 0.13 | 45.3 ± 4.9 | 2 |
Rabbit40 | 2.33 ± 0.21 | 11.6 ±1.5 | 3 |
Modified from reference 17.
Placental structure changes significantly between the first and third trimesters to accommodate increasing fetal oxygen demands (Table 5.2).22,27,41–44 Thus, placental weight and exchange area increase with gestation in parallel with the growing fetus. Although placental and fetal weights both increase throughout pregnancy, fetal weight accelerates during the third trimester, resulting in a 50% decrease in placental:fetal weight ratio from 0.22 to 0.14.16 The growth of the placental villous structure and thus the increase in vascularity results in increased exchange area and decreased distance of exchange, culminating in a 30-fold increase in absolute diffusing capacity between 10 and 40 weeks’ gestation.44,45 The diffusion distance is decreased to 3.5 μm in the normal near-term placenta through proximity of fetal vasculature to the trophoblastic membrane, resulting from the reduction in thickness of the stroma and trophoblast layers.41
Table 5.2 Placental structural changes with gestational age in humans
First trimester | Third trimester | Direction of change | |
---|---|---|---|
Fetal weight (kg) | 0.15 | 3.3–3.5 | ↑ |
Placental weight (g) | 70 | 500–600 | ↑ |
Villi exchange surface area (m2) | 1.5 | 12–14 | ↑ |
Fetal capillary surface area (m2) | 1 | 11 | ↑ |
Total villous volume (cm3) | 35 | 210 | ↑ |
Mean villous diameter (um) | 90 | 55 | ↓ |
Number of microvilli (×106/cm2) | 600 | 1200 | ↑ |
Villous membrane thickness (um) | 10 | 2 | ↓ |
Fetal capillary volume (cm3) | 4 | 48 | ↑ |
In addition to placental structure, the diffusion of oxygen across the placenta is influenced by the direction of maternal and fetal blood flows. Human, primate, sheep and dog placentas are concurrent exchangers of oxygen and nutrients because the incoming maternal and fetal arterial blood both flow in the same direction (Figure 5.4A).17,20,46 The fetal blood oxygen in the placenta equilibrates to the maternal venous oxygen, leading to a relatively low concentration gradient in the blood exiting the intervillous space where fetal and maternal blood PO2 in the placenta is 28.7 and 29.9 mmHg, respectively.21 However, the umbilical venous PO2 is ~10–15 mmHg lower than uterine venous PO2 (Figure 5.3) due to a combination of placental vascular shunts (20–36% arterial blood bypassing the exchange area and anastomosing with the venous return), regional inequalities/uneven distribution in maternal and fetal blood flow over the length of the placenta and inequalities in placental membrane characteristics influencing diffusion capacity.16,17,47,48 Compared to PO2, the SO2 of umbilical and uterine veins are relatively similar.
Figure 5.4 (A) Humans, monkeys, sheep and dogs have concurrent placental circulations where maternal and fetal blood flow in the same direction and fetal venous levels approximately equilibrate to maternal venous levels. (B) In contrast, guinea pigs and rabbits have placentas that operate as counter-current exchangers where maternal and fetal blood flows in the opposite direction. In the counter-current placenta. The normal mean late gestation PO2 values (mmHg) for arterial and venous vessels for both types of placental gas exchange systems based on respective animals are listed (A and B).17 UtV = uterine vein; UtA = uterine artery; UV = umbilical vein; UA = umbilical artery.
In contrast, rabbits and guinea pigs have counter-current exchange in their placentas, whereby the maternal and fetal blood flow in opposite directions (Figure 5.4B).17,20,46 Although, the counter-current exchange is more efficient at transporting of oxygen, the fetal umbilical vein PO2s are relatively low and do not equilibrate to the maternal arterial blood due to lower maternal placental blood flows. Thus, a counter-current system provides more effective gas exchange for the same amount of flow and results in closer equilibration between fetal umbilical venous and maternal uterine arterial PO2 compared to a concurrent exchanger. In a counter-current exchanger, the umbilical venous PO2 is actually ~10 mmHg higher than uterine venous PO2. Higher efficiency of the counter-current placenta is evidenced by higher DpCO in guinea pigs and rabbits than humans (predicted), sheep, dogs and monkeys. The DpCO in near-term guinea pigs is ~2.92 ml/min/mmHg/kg, which is 5–6-fold higher than those with concurrent exchange placentas.35,49 The number of cell layers forming the placental exchange membrane that oxygen and nutrients must cross also varies amongst species (Table 5.1): guinea pig (two cell layers), rabbit (three), dog (four), sheep (five), monkey (three) and human (three).17 Thus, the combination of a counter-current system with fewer cell layers between the fetal and maternal circulations results in the most efficient mode of gas exchange.
Fetal health is placed at risk in conditions that affect placental structure. It is predicted that placental diffusing capacity decreases as a result of thickening of placental membranes due to the associated increase in the diffusion distance in conditions such as gestational diabetes mellitus,50 syphilis51 and in the setting of intrauterine growth restriction (IUGR).41 Incomplete uterine spiral artery remodelling forms the basis of placental-derived IUGR in which the placenta also has reduced growth and exchange area characterized by reduced villous volume, villous surface area and fetal capillary vascularization of the microvilli.52,53 Currently, placental structure can only be examined histologically after delivery. Doppler ultrasound interrogation of the uterine and umbilical vessels provides some information about the resistance of the downstream placenta. However, the development of further techniques such as magnetic resonance imaging (MRI) methods for measuring both oxygen saturations and blood flows in the uterine and umbilical vessels could advance the detection of placental dysfunction and provide an opportunity for interventions to improve fetal outcome.
Maternal and Fetal Oxygen Partial Pressures
Oxygen transfer between the mother and fetus is dependent on the difference in PO2 between the two circulations, thus changes in either maternal or fetal PO2 can impact oxygen transfer. Ex vivo placental perfusion studies are useful because changes in PO2 can be isolated from in vivo compensatory mechanisms. However, the magnitude of change observed ex vivo is relatively larger than in vivo because of the lack of compensatory mechanisms. One such study in sheep and rabbits (controlled fetal umbilical blood flow rate and PO2 and maternal inspired oxygen) showed that placental oxygen transfer is affected by changes in fetal umbilical arterial PO2.54 A 13% rise in oxygen transfer rate resulted from a 20% reduction of fetal umbilical arterial PO2 from normal levels of ~15 mmHg. Although the oxygen transfer rate was influenced by umbilical arterial PO2, umbilical blood flow was the stronger contributor to alterations in both the oxygen transfer rate and umbilical venous PO2. This ex vivo study concluded that placental oxygen transfer changes by ~10% if the following variables change from normal levels: 20% change in maternal arterial PO2 or 20% change in umbilical arterial PO2 or a 14% change in umbilical blood flow (Figure 5.5). Fetal arterial PO2 levels depend on the amount of placental oxygen transfer, umbilical venous PO2 and thus fetal oxygen consumption. Therefore, normal variations in oxygen transfer may be driven by changes in fetal arterial PO2 because a 3 mmHg change in umbilical arterial PO2, which is within normal day-to-day variation, results in a 10% change in oxygen transfer rate to the fetus.
Figure 5.5 Factors affecting placental oxygen transfer based on ex vivoperfusion of sheep and rabbit placenta. For example, an increase of 20% in maternal arterial PO2 or 14% in umbilical blood flow or a decrease of 20% in umbilical arterial PO2 from normal are all equivalent in bringing ~10% increase in oxygen transfer to the fetus and vice versa.54
A small reduction in maternal arterial PO2 from normal levels causes a modest reduction in SO2 as the oxygen dissociation curve is relatively flat above 70 mmHg, with maternal arterial oxygen saturations of 90% or more (Figure 5.6). A decrease in maternal arterial PO2 to 50 mmHg lowers placental oxygen transfer by 40–50% (umbilical venous PO2 ~23 mmHg) whereas an increase in maternal arterial PO2 to 340 mmHg increases the oxygen transfer rate by 40% and umbilical venous PO2 by ~25% in ex vivo perfused placenta.54 Having the mother inspire 100% oxygen can increase maternal arterial PO2 to ~300 mmHg, which would only slightly increase the maternal blood SO2 as it is already near 100% saturated in normal conditions when inspiring room air. However, high maternal arterial PO2 does increase fetal blood PO2 by ~5 mmHg in normal cases. Women undergoing caesarean section inspiring 100% oxygen had increased umbilical venous and arterial PO2 of 8 and 5 mmHg, respectively, but the umbilical veno-arterial difference did not change.55 Similarly, no significant change in fetal oxygen consumption was observed in an acute fetal sheep experiment with pregnant ewes inspiring 100% oxygen.56 In a normal fetus maintaining normal oxygen consumption, a 5 mmHg change in PO2 may not be significant. However, in the setting of fetal hypoxaemia, such a change could be of clinical therapeutic importance, for example by improving the oxygen supply to the IUGR fetus.57–59However, maternal hyperoxygenation does not improve nutrient supply to the utero-placental unit and its effect on the fetal metabolism remains to be investigated.
Figure 5.6 Oxyhaemoglobin dissociation curve (ODC). A leftward shift indicates higher oxygen affinity by haemoglobin (e.g. fetal blood) and a rightward shift infers lower oxygen affinity (e.g. adult blood). Haemoglobin oxygen affinity is affected by four factors: temperature, 2,3-DPG, PCO2 and [H+]. Increases in these four factors result in a rightward shift of the ODC, meaning reduced haemoglobin oxygen affinity, while more readily releasing bound oxygen. A decrease in these four factors results in a leftward shift of the ODC. Adapted from reference 68.
Significant reductions in maternal arterial PO2 result in low fetal umbilical SO2 levels resulting from a reduction in placental oxygen transfer and fetal oxygen delivery. Maternal hypoxaemia due to cyanotic heart disease, respiratory disease or high altitude is expected to result in lower oxygen transfer and reduced umbilical venous PO2. Although there are no fetal blood gas values available from pregnancies complicated by maternal cyanotic heart disease, the live birth rate in this population is 40–45% and 10–15% if maternal arterial SO2 is 85–90% and <85%, respectively.60–62 These findings suggest that fetal health is significantly compromised throughout pregnancy due to reduced placental oxygen transfer and thus fetal oxygen delivery. Chronic pulmonary disease, like asthma and cystic fibrosis, and acute respiratory infections, like bronchitis or pneumonia, in pregnancy can result in maternal and fetal complications due to chronic maternal hypoxia. Furthermore, maternal smoking and significant anaemia can impair maternal oxygen supply to the utero-placental unit and the fetus. These maternal complications are associated with an increased risk of premature birth, IUGR and stillbirth.61 Several animal models of maternal hypoxia in late gestation have been developed to tease apart the specific effects of reduced fetal oxygen delivery on fetal and organ development.63–67 High altitude pregnancies will be discussed later in more detail.
Maternal and Fetal Haemoglobin–Oxygen Affinity
Before haemoglobin oxygen affinity is discussed in detail, it is necessary to be familiar with the oxyhaemoglobin dissociation curve (ODC; Figure 5.6). The ODC describes the relationship between the PO2 in the surrounding tissue/environment on the x-axis and the amount of haemoglobin saturated with or bound by oxygen (SO2, %) on the y-axis and is sigmoid in shape. As such the curve describes the behaviour of haemoglobin in terms of its affinity to oxygen, which is low at low PO2 and high at high PO2; however, there is a notable plateau of the curve at higher PO2 whereby the haemoglobin becomes fully saturated with oxygen without any further increase in SO2 beyond 100%. If the surrounding tissue has low PO2 (e.g. muscle tissue), haemoglobin in red blood cells will donate oxygen molecules to the surrounding tissue and if the surrounding tissue has high PO2 (e.g. alveoli in lungs), the haemoglobin will bind oxygen and become saturated. There are several factors that can shift the curve leftwards or rightwards, as indicated by the arrows in Figure 5.6, which are of significance in understanding placental gas exchange.
The haemoglobin molecule is a tetramer and its composition of subunits in adult (HbA) and fetal (HbF) blood is different. The protein structure of HbF consists of two α and two γ subunits, including their four heme groups in comparison to two α and two β subunits in HbA. Importantly, HbF does not bind 2,3-disphosphoglycerate (2,3-DPG) as well as HbA (right shift of HbA), resulting in decreased affinity for oxygen by HbA. It is the less positively charged γ subunit (fetal) in place of the β (adult) which makes the HbF less attractive to 2,3-DPG and results in HbF higher affinity for oxygen than HbA. This difference can be best understood using an ODC. The HbF ODC curve is shifted left, therefore given the same PO2, HbF will be more saturated (higher SO2) than HbA. The higher affinity of HbF for oxygen (compared to HbA) promotes oxygen transfer from maternal blood (a mixture of oxygenated and deoxygenated blood due to placental oxygen consumption) to the fetal chorionic villi.16,17 The P50, which is the PO2 at which blood has 50% SO2 is ~27 mmHg in maternal blood at normal conditions (temperature 37°C, pH 7.40 and PCO2 40 mmHg), whereas in the fetus, where the ODC is shifted left, P50 is ~19 mmHg (Figure 5.6).16,20 About 70% of the newborn’s haemoglobin is fetal at birth, which decreases to <5% by about 6 months of age.69 HbF is present in minute quantities in adults (<1% of total haemoglobin).17
The lower oxygen affinity of HbA compared with HbF also helps the fetus take up oxygen, as HbA more readily gives up its bound oxygen in the placenta. A good illustration of the impact of the two forms of haemoglobin is the lack of any SO2 gradient between umbilical venous and uterine venous blood despite fetal umbilical venous PO2 being ~10 mmHg lower than uterine venous blood (Figure 5.3).
The affinity of haemoglobin for oxygen is affected by four main factors: temperature, 2,3-diphosphoglycerate (2,3-DPG) concentration, PCO2 and pH/hydrogen ion concentration, [H+]. An increase in the levels of any of these four factors results in a rightward shift of the ODC, i.e. reduced haemoglobin oxygen affinity, resulting in haemoglobin more readily releasing bound oxygen. A rightward shift is needed in the setting of exercise where the muscle tissues require more oxygen. A decrease in the levels of these four factors results in a leftward shift of the ODC. A leftward shift indicates higher haemoglobin affinity for oxygen, a feature that is helpful, for example, at the alveoli–capillary interface in the lungs where it promotes the uptake of oxygen by the blood. In placental gas exchange, [H+] and PCO2 levels are most important because of their effect on the oxygen affinity of HbF.
Two important phenomena, the Bohr and Haldane effects, take place at the placental exchange interface and facilitate the release of oxygen by maternal blood and uptake of oxygen by fetal blood. The Bohr effect involves [H+], while the Haldane effect is related to PCO2. Fetal blood has higher levels of [H+] and PCO2 than maternal blood, and so [H+] and CO2 diffuse down their concentration gradients across the placenta and into maternal blood, making the maternal intervillous blood more acidotic (low pH) and hypercarbic (high CO2). Increasing levels of [H+] and CO2 in maternal blood shift its ODC to the right, resulting in maternal haemoglobin releasing oxygen more readily. Meanwhile, as fetal blood is losing [H+] and CO2, the fetal blood ODC shifts left, increasing oxygen uptake by HbF. About 8% of oxygen exchange at the placenta is associated with this phenomenon known as the double Bohr effect where the fetal and maternal blood ODC move in opposite directions.70
The Bohr effect results in increased oxyhaemoglobin levels in fetal blood and decreased oxyhaemoglobin levels in maternal blood, implying greater deoxyhaemoglobin levels in maternal blood. CO2 binds more readily with deoxyhaemoglobin relative to oxyhaemoglobin, resulting in exchange of CO2 from fetal to maternal placental blood. About 46% of CO2 exchange in the placenta is associated with this phenomenon, known as the Haldane effect.70 CO2 in blood is found in three forms: dissolved, bicarbonate ions and in combination with proteins, with the majority (~90%) found as bicarbonate ions.43 Catalytic hydration of CO2 by carbonic anhydrase enzyme to carbonic acid results in formation of a hydrogen ion, H+, (Equation (5.4)) that shifts the ODC curve rightward and increases the affinity of maternal haemoglobin for CO2. In contrast, the HbF ODC moves left with the efflux of CO2 and [H+] and more binding of oxygen in the placenta. Hyperventilation is one of the maternal adaptations to pregnancy, resulting in lower PCO2 in maternal blood, supporting the transfer of CO2 down its concentration gradient from fetal to maternal blood at the placenta. Thus, there is a simultaneous exchange of oxygen from maternal to fetal blood and carbon dioxide from fetal to maternal blood.

Maternal and Fetal Blood Oxygen-Carrying Capacity
In addition to the structural differences in haemoglobin between mother and fetus, there are also differences in haemoglobin concentrations, with fetal blood having a higher haemoglobin concentration than maternal blood. Maternal cardiovascular changes that accompany pregnancy include ~40% increase in maternal plasma volume leading to an increase in blood volume71 and 25% increase in erythrocyte mass over the course of gestation.72 In human pregnancies, maternal haemoglobin decreases from ~14 g/dl before pregnancy to ~11 g/dl in late gestation,71 whereas fetal haemoglobin increases from a mean of ~9 g/dl around 10 weeks’ gestation to 14 g/dl at 22 weeks and 17 g/dl near term.16,32 Given these haemoglobin concentrations, blood oxygen-carrying capacity, which is the maximum quantity of oxygen that can bind with haemoglobin, can be calculated using the following formula:
where 1.36 is the amount of oxygen in ml bound to a gram of haemoglobin. Using this formula, it is evident that the fetal blood oxygen capacity (~23 g/dl) is greater than maternal (~15 g/dl) during late gestation (Figure 5.3). Higher blood oxygen capacity leads to higher blood oxygen content. The fetal umbilical vein SO2 is 70–75%; however, due to the higher concentration of haemoglobin, fetal blood oxygen content is about the same as maternal arterial blood oxygen content, despite the higher SO2 of 98% in the mother (Figure 5.3). Placental oxygen exchange is facilitated by an increase in either maternal or fetal oxygen capacity/content.73 Thus, maternal and fetal placental blood SO2 can influence oxygen exchange.
Maternal and Fetal Placental Blood Flow
Fetal (umbilical) and maternal (uterine) placental blood flow rates are the most critical determinants of placental gas exchange, because the rate of oxygen exchange positively correlates with fetal and maternal placental blood flow.73–76 A good example of this relationship can be observed during various stages of labour, where there are transient reductions in spiral artery blood flow during uterine contractions that reduce oxygen delivery to the fetus, especially in the later stages of labour, as indicated by decelerations in the fetal heart rate.17 Furthermore, uterine artery blood flow is positively correlated with birth weight.77 Maternal and fetal placental blood flow in normal, growth-restricted and high-altitude pregnancies will be presented in this section.
Normal Maternal and Fetal Placental Blood Flow
There is a profound increase in uterine artery blood flow across pregnancy from a pre-pregnancy flow of 20–50 ml/min to 700–1000 ml/min in humans78–84 and 1200–1500 ml/min in sheep85–87 in late gestation. In humans this is supported by an increase in maternal cardiac output until a plateau is reached in the third trimester (from ~4 L/min in pre-pregnancy to ~7 L/min in late gestation88). As such, uterine blood flow accounts for <1% of the maternal cardiac output pre-pregnancy89 and this proportion increases substantially due to the ~20-fold increase in uterine artery blood flow to account for ~15% of the maternal cardiac output in late gestation. The increase in maternal cardiac output is achieved through an increase in stroke volume and heart rate. However, the initial increase in stroke volume reaches its peak by the early stages of the second trimester and then plateaus, even declining slightly later in pregnancy. Subsequent increases in cardiac output are sustained by the gradual increase in heart rate throughout pregnancy.71
The relatively large range of uterine blood flow reported by different studies may reflect wide variations in normal physiology, as well as differences in the methods used to measure this parameter. Approaches to measuring uterine blood flow include invasive haemodynamic assessment based on the Fick principle, as well as a range of Doppler techniques, including flow probes surgically placed around vessels and non-invasive ultrasound approaches. Each of these techniques have their strengths and weaknesses. For example, Doppler ultrasound approaches frequently assume uniform mean velocity of blood flow across the vessel lumen, while the laws of fluid dynamics decree that flow in a cylinder has the highest velocities at the centre. Techniques such as flow probes or calculations made using measurements of arterial and venous blood gases and oxygen consumption are likely to overcome the limitations of non-invasive ultrasound. However, these methods require invasive surgical preparation and thus are limited to use in animals. Any measurement collected with the use of sedation or anaesthesia may be confounded by the drug altering placental haemodynamics. These techniques have not been used routinely in humans due to the associated risks to the mother and fetus. Cine phase-contrast magnetic resonance imaging (PC MRI) overcomes the limitations of ultrasound as it accounts for the different flow velocities across the vessel lumen, and yet this is an entirely non-invasive technique that can be used to study the maternal and fetal placental circulations without sedation. Hence, PC MRI has become the non-invasive gold standard for vessel blood flow quantification.90 We have employed PC MRI in late gestation human pregnancies and observed mean uterine artery blood flow of ~1100 ml/min, similar to the values obtained with invasive techniques.91
Fetal umbilical vein (UV) blood flow increases significantly throughout gestation in normal pregnancies (Table 5.3). However, when indexed to fetal weight, UV flow decreases gradually with gestation (more so in humans than in sheep).12,27,92,93 UV flow in sheep fetuses is higher than in human fetuses and in late gestation, UV flow accounts for 38–45% of combined ventricular output (CVO) in sheep but only 20–30% in humans (Table 5.3).15,94,95 The lower fetal–placental circulation in human fetuses may support greater distribution to the brain (24 vs 3.5% of CVO in humans vs sheep) as human fetal brains weigh significantly more (350 vs 65 g) in late gestation.94 It is speculated that the lower UV flow seen in humans compared with sheep is tolerated because of the higher haemoglobin concentration, and therefore higher oxygen-carrying capacity of human fetal blood (Table 5.4).94 To accommodate the increase in UV flow, the UV increases in diameter from 4.1 mm at 20 weeks to 8.3 mm at 38 weeks gestational age in normal human pregnancies.12 Fetal UV blood flow in humans measured by MRI is ~135 ml/min/kg in late gestation and accounts for ~30% of the CVO.15,96,97 The UV blood flow reported by ultrasound studies underestimates those from MRI studies likely due to errors in estimating vessel cross-sectional area and assumption of mean flow velocity across the vessel lumen to derive blood flow measurements.98 Despite ultrasound-associated errors in flow measurements, the data is included here to appreciate the longitudinal changes in fetal–placental blood flow during pregnancy (Table 5.3).
Table 5.3 Changes in umbilical vein blood flow throughout pregnancy
Human12 | Sheep92 | Human12 | Sheep92 | Human12 | Sheep92 | |
---|---|---|---|---|---|---|
Early | Mid | Late | ||||
Term | 40 weeks | 150 days | 40 weeks | 150 days | 40 weeks | 150 days |
Age measurement made | 20 weeks | 73 days | 30 weeks | 116 days | 38 weeks | 146 days |
ml/min | 60 | 62 | 170 | 449 | 370 | 796 |
ml/min/kg fetal weight | 130 | 232 | 117 | 230 | 105 | 221 |
%CVO | 3295 | 47 | 2795 | 46 | 2095 | 41 |
%CVO = umbilical blood flow as a percentage of combined ventricular output. Note: The UV flow in human fetuses was measured using ultrasound, whereas in the sheep it was measured using radionuclide-labelled microspheres.
Table 5.4 Human and sheep fetal umbilical vein and artery blood gas in late gestation
Human Fetal (term 40 weeks) | Sheep fetal(term 150 days) | |||
---|---|---|---|---|
Umbilical vein | Umbilical artery | Umbilical vein | Umbilical artery | |
PO2 (mmHg) | 30 | 20 | 33 | 23 |
PCO2 (mmHg) | 41 | 52 | 39 | 47 |
pH | 7.347 | 7.277 | 7.396 | 7.342 |
Hb (g/dl) | 16.0 | 16.0 | 10.8 | 10.8 |
Hct (%)* | 48 | 48 | 33 | 33 |
SO2 (%) | 70 | 50 | 83 | 55 |
O2 capacity (ml/dl) | 21.8 | 21.8 | 14.8 | 14.8 |
O2 content (ml/dl) | 15.2 | 10.9 | 12.2 | 8.1 |
Fetal oxygen delivery (ml/min/kg fetal weight) | ~20 | – | ~25 | – |
Pregnant women are often recommended to rest in a left lateral decubitus position because being in the supine position can result in inferior vena caval compression due to the substantial weight of the uterus and fetus in late pregnancy.108,109 Such compression reduces venous return to the heart and thus reduces maternal cardiac output. Reduced placental venous outflow on the maternal side may also increase pressure in the intervillous space, which would increase placental vascular resistance due to possible compression of fetal–placental vasculature and consequently decrease fetal placental blood flow.16,110 Although, these changes may be transitory and dependent on the duration of maternal position, there is evidence for poor fetal outcomes in supine sleepers and interventions to prevent supine sleeping have been successful.111 This is an important area for research.112
Maternal and Fetal Placental Blood Flow in the Setting of Complicated Pregnancies
In IUGR resulting from utero-placental vascular insufficiency, the placenta is characterized by abnormal villous development, reduced villous density and intervillous space size, smaller placental and villous tree size (poor villi branching), increased path length of diffusion resulting in reduced fetal oxygen delivery, but a relatively smaller reduction in fetal oxygen consumption indexed to fetal size compared to age-matched controls.113,114 These structural changes correlate with placental haemodynamics of significantly reduced uterine and umbilical blood flows, resulting in reduced fetal and placental weights and oxygen delivery and consumption.97,115–120 Furthermore, uterine blood flow may also be decreased in the setting of maternal vascular disease/complex heart disease and pre-eclampsia during pregnancy, although few studies have measured uterine blood flow in these populations. Recent findings regarding pregnancy outcomes in mothers with single-ventricle hearts121 and late-onset pre-eclampsia77 suggest there is adequate uterine blood flow in most of these conditions to support the growing fetus. However, significantly reduced uterine blood flow has been reported in early-onset pre-eclampsia pregnancies compared to controls, resulting in reduced placental oxygen transfer and compromised fetal growth and health.77 Furthermore, maternal cyanosis and inability to augment cardiac output are known to be the most significant predictors of poor fetal growth in the setting of maternal heart disease.122 As discussed above, placental oxygen exchange is strongly correlated with uterine artery blood flow.16,76,123
One longitudinal study quantified the changes in uterine artery blood flow using ultrasound in normal human pregnancies and in those affected by mild IUGR (birth weight between 10th and 3rd centile).81 The average birth weight of IUGR newborns was ~23% lower (2634 g) than normal controls (3429 g). Uterine artery blood flow was significantly reduced in IUGR subjects and the difference was more pronounced in late second trimester and onwards (Table 5.5). Blood flow in a vessel is dependent on vessel size and flow velocities, and in the IUGR group, uterine artery diameter was reduced throughout pregnancy, with greater differences in late second trimester and onwards. However, uterine artery blood flow indexed to fetal weight decreased with gestation and was not significantly reduced in IUGRs compared to controls throughout pregnancy (Table 5.5). Remodeling of the uterine spiral arteries in early pregnancy, which transforms them from high resistance, low flow to low resistance, high flow vessels to support the oxygen and nutrient demands of the growing fetus throughout pregnancy, takes place in the decidua and the inner regions of the myometrium,124–126 whereas the changes in diameter measured in this study were in uterine artery sections outside the myometrium. The upstream reductions in diameter and flow may be indicative of possible associated downstream deficits in remodelling of spiral arteries resulting in a high-resistance utero-placental circulation. Furthermore, low utero-placental blood flow is associated with pregnancy pathologies like pre-eclampsia, placental infarcts, unusually small placental villi and high number of syncytial knots.127 Pregnancies complicated by IUGR and/or pre-eclampsia are also associated with reduced maternal cardiac output and increased peripheral vascular resistance compared to controls.128–130
Table 5.5 Changes in uterine artery blood flow in humans throughout pregnancy
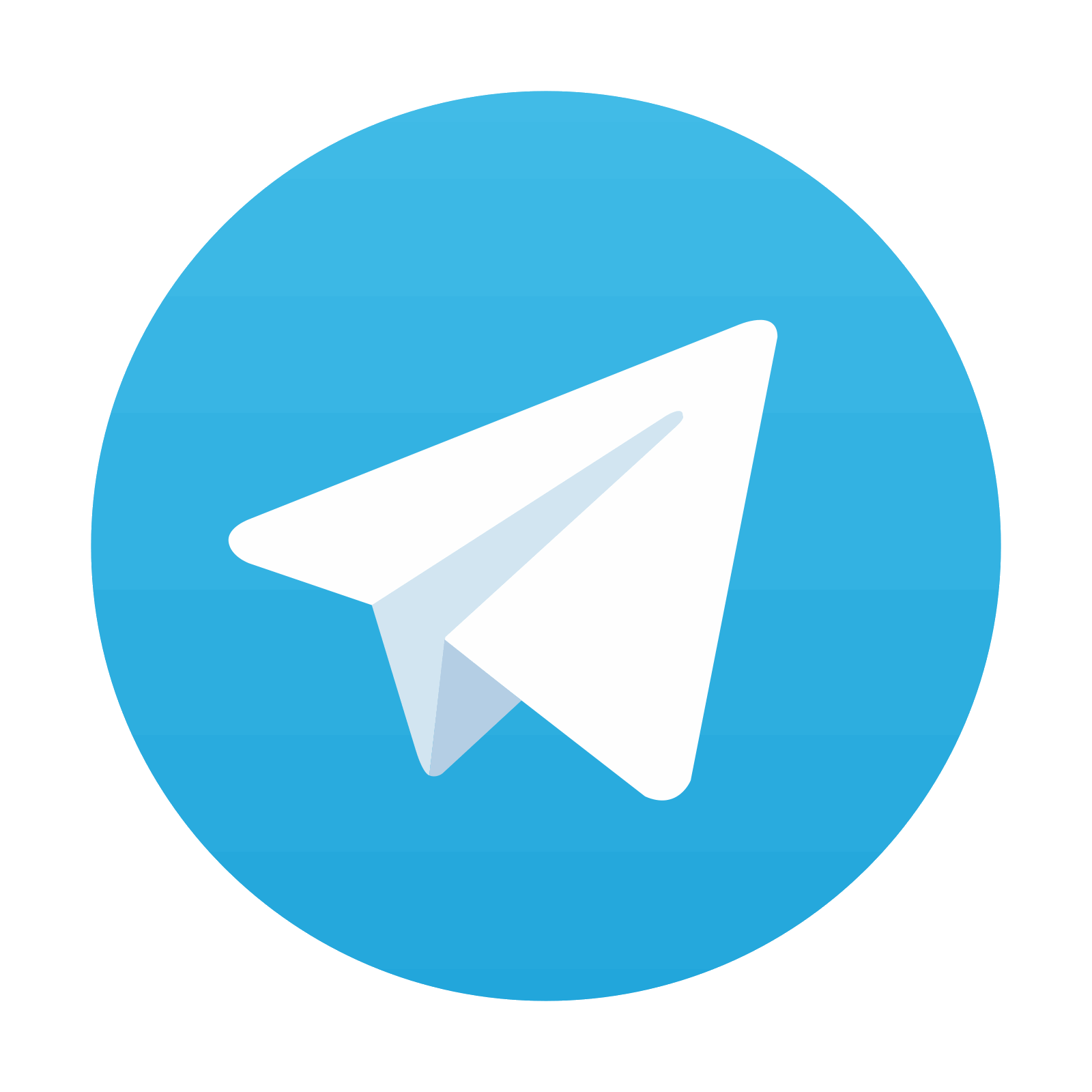
Stay updated, free articles. Join our Telegram channel
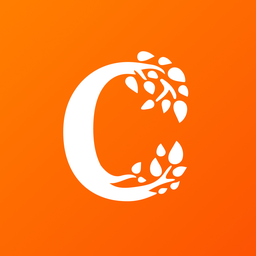
Full access? Get Clinical Tree
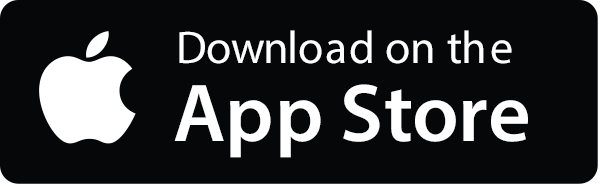
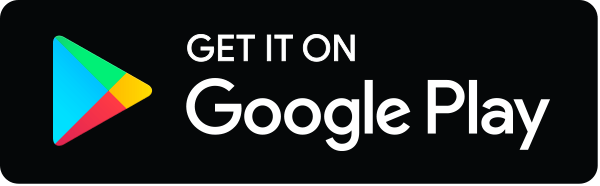