Fig. 6.1
As the solute (including the cryoprotectant) concentration of a solution increases (x axis), the cooling and warming rates (warming rates shown here on y axis) necessary to maintain an ice-free state decrease, and this decrease continues until no measurable ice forms in the solution and occurs at very low rates of temperature change (<1 °C per minute)
Chemical and osmotic toxicity of cryoprotectant solutions are a far greater concern for vitrification procedures. This is because solutions used for freezing (versus vitrification) are much less concentrated at the temperature associated with initial cell and tissue exposure. The good news is that these problems can often be overcome. Adding and removing the cryoprotectants in several steps, and also using of osmotic buffers such as non-permeating sugars during cryoprotectant removal, can overcome excessive osmotic stress [29]. One caveat to using this approach is that the exposure time to the cryoprotectants will be longer and may exacerbate chemical toxicity. Adding and removing cryoprotectants at cold temperatures (i.e., 4 °C or lower), at least during the steps where higher concentrations are relevant, has the potential to reduce chemical toxicity. This approach may exacerbate osmotic stress, however, as the permeability of cryoprotectants to cells is usually much lower at these temperatures compared to water [25]. Another approach to reducing osmotic and chemical toxicity is to expose the cells to high values of these stresses for short periods of time. This approach is commonly utilized in current methods to vitrify oocytes and embryos, as the steps associated with exposure to the vitrification solution before cooling and during warming are very short (often less than 1 min). Such an approach will be more difficult for tissue, however, as the time necessary to concentrate the intracellular cryoprotectants sufficiently to maintain a stable vitreous state during the final step of cryoprotectant addition depends upon the size and geometry of the sample. This simply cannot be accomplished for tissue pieces on the same time scale as it is done for oocytes and embryos. As a result of these challenges inherent with tissue vitrification, thoughtful experiments must be designed and conducted in order to achieve effective vitrification.
A final challenge that we wish to discuss is that associated with the fracturing of a vitrified solution. The assumed need to cool and warm as fast as possible to successfully vitrify has led many investigators to plunge relatively large samples (e.g., cryostraws and cryovials) directly into liquid nitrogen to facilitate rapid cooling. While this approach does maximize the cooling rate, this approach can result in the generation of excessive thermomechanical stress within the vitrified sample, and fracturing of the vitrified glass is often seen, and occurs to relieve this stress [34]. The problem with glass fracturing is that the tissue is also prone to fracturing, and this can result in irreversible damage. One way to avoid the buildup of this stress is to slow down the cooling and warming of the sample. This apparent paradox leaves many confused. However, as we just described, rapid cooling and warming is not necessary to achieve and maintain a vitreous state if other variables in the system are properly designed.
As one can gather from the above discussion, vitrifying larger samples, such as tissue, becomes rather complicated due to the inherent limitations that the size of the material imposes on the system. However, it is our belief that with thoughtful experiments designed to understand the sensitivities of the material that one wishes to vitrify, it will be possible to engineer procedures that account for these sensitivities and result in a method that successfully preserves the viability of the biomaterial.
Development of Ovarian Tissue Vitrification Based on Cryobiological Principles
Vitrification has benefits independent from the ability to prevent lethal ice formation in a sample. From a practical standpoint, it is often a faster means to get samples into cryogenic storage. For isolated cells like oocytes, a procedure on one to two oocytes can be completed in approximately 12 min. On the contrary, because freezing cools the cells very slowly (i.e., 1 °C per minute between −5 and −35 °C), a freezing procedure usually takes more than an hour.
As with any multiparameter procedure, there are literally countless numbers of ways to vitrify biological material. The steps to vitrify a sample include incubating the biomaterial in a solution containing cryoprotectants, loading the sample into or onto a support system, cooling that system, holding the system in a long-term storage vessel, warming the sample, diluting the cryoprotectant solution, and then utilizing the sample in the intended way. Since each of these steps could be done in any one of a number of ways, the possible number of combinations of these parameters is theoretically infinite. This variety of combinations of parameters is reflected in the fact that more than 40 vitrification procedures have been developed and tested on human ovarian tissue throughout the years [1], the majority of protocols having been developed for oocyte or embryo vitrification, but not optimized for the complexity of cell types within ovarian cortical tissue that differ in cryoprotectant uptake and osmotic tolerance.
While it is likely that any number of methods could work equally effectively, it is also likely that more methods would fail than succeed. The difficulty, then, is to sort through all of the possible choices of parameters to find the right combination. We have approached this task in our efforts to vitrify nonhuman primate ovarian tissue by using some fundamental cryobiological principles to narrow the possible choices and, within that more limited sampling space, identified better choices based upon empirical testing.
One of the most important design restrictions upon which we imposed ourselves was that the sample had to be held inside of a truly closed system; closed in this context means that the sample is isolated from its environment during storage. We achieved this goal by using a straw that is large enough to hold small tissue pieces yet can be hermetically sealed. Using this vessel imposed restrictions on the other parameters, most noteworthy the achievable rates of temperature change during cooling and warming.
Since the straw we have chosen is much larger than the devices currently used to vitrify cells in embryology laboratories, we were limited to cooling and warming rates no greater than a few hundred °C per minute. As a result, this required us to develop vitrification solutions that would form stable glasses at such cooling and warming rates. As mentioned above, this is achievable, contrary to much of the current thinking.
Using a larger system does have some advantages, such as easier handling and labeling. The disadvantages include the use of higher concentrated solutions, with presumably higher toxicity, as discussed. However, we felt that, with enough effort, we would be able to overcome this challenge and develop a vitrification method that would form a stable glass and also have limited toxicity to primate ovarian tissue, allowing its use in a newly designed system.
Another advantage of having a larger system is that it is quite easy to observe ice formation during cooling and warming. We took advantage of this fact when designing our vitrification solutions and confirmed the absence of ice during cooling and warming by using a sensitive thermal analysis (differential scanning calorimetry).
Relatively few compounds have been identified that have cryoprotective properties and can be used as cryoprotectants. In the area of assisted reproduction, these include dimethyl sulfoxide (DMSO), ethylene glycol, propylene glycol, glycerol, and sucrose. All of these compounds except for sucrose fall into a class known as permeating cryoprotectants since they are able to diffuse across the cell membrane and enter the cytoplasm. In nearly all instances known, a cryopreservation solution must have at least one permeating cryoprotectant. Since vitrification solutions contain a relatively high concentration of cryoprotectants, a combination of two or more of these compounds are usually utilized, in an effort to reduce the specific toxicity of each single compound.
In one of our early experiments, we compared two vitrification solutions, one containing a combination of DMSO and ethylene glycol and the other containing a combination of glycerol and ethylene glycol. In order to determine the appropriate concentration of each of the compounds, we tested solutions containing an increasing concentration of the combination of each. Our goal was to have a solution with just enough of the solutes to form a stable glass in the straw system – too much would exacerbate chemical toxicity, and too little would facilitate ice formation. It was determined that with the combination of DMSO and ethylene glycol, a total concentration of 51 % by weight would suffice, and with the glycerol-ethylene glycol combination, 53 % was sufficient.
When testing these solutions, we witnessed a phenomenon that rarely occurs and goes against common assumptions. At these concentrations, while the solutions themselves remained vitreous during cooling and warming, the pieces of tissue actually froze during warming! It is often assumed that, because the cytoplasm of cells contains such a high concentration of proteins and other macromolecules, the cytoplasm is much more likely to vitrify than the surrounding solution. While this is probably true in a general sense, it is not an absolute fact, as we observed (also, see the discussion in [13]). We were able to overcome this problem by simply increasing the overall solute concentration by 1 % to a total of 54 % when using the glycerol and ethylene glycol combination. With the slightly higher concentration of solutes, both the solution and the tissue appeared to remain vitreous throughout both the cooling and warming process (Fig. 6.2).

Fig. 6.2
Top: Devitrification of macaque ovarian tissue contained inside a heat-sealed high security plastic straw is observed when the concentration of permeating cryoprotectants (52 %) is not optimal. Bottom: Successful vitrification of macaque ovarian tissue in a closed system upon both cooling and warming occurs with optimal concentration of permeating cryoprotectants (54 %) (See Ting et al. [38] for details)
We also tested the addition of synthetic polymers to the vitrification solutions, to determine their effect on the tissue after cooling and warming. The particular polymers were chosen for their ability to enhance vitrification at slow cooling and warming rates. Based upon the molecular configuration of these polymers, it is believed that they either directly interact with ice crystals, preventing their growth, or they interact with inherent nucleating agents that are often found in solutions, reducing the ability of ice crystals to form. Whatever their mechanism, we found that the addition of these polymers to the vitrification solution resulted in better preservation of the tissue. Because of their presumed mechanism of action, these polymers are only included in the vitrification solution (i.e., not the cryoprotectant loading or equilibration solutions) used in the last step prior to cooling of the tissue.
In each of the experiments that we conducted, we were able to identify levels of parameters that resulted in improvements in the experimental outcomes. We progressed in an incremental fashion, choosing the best parameter levels to include in future experiments, where other parameters were assessed. In the end, we have developed a vitrification method that met our design criteria – having a closed system that is easy to use and insures that the sample remains vitreous throughout the entire procedure. The good news is that the tissue, having been vitrified using this system, maintains a high level of viability. The viability of the tissue remains slightly less than the non-cryopreserved tissue, as measured by our experimental endpoints, suggesting that further improvements might be achievable. Nevertheless, we feel that, by applying good cryobiological principles and designing careful experiments, we were able to make significant progress toward the development of a very good vitrification method for nonhuman primate ovarian tissue that can eventually be applied to human tissue.
Endpoints of Vitrification Success
The gold standard and ultimate assessment for the success of a vitrification protocol for ovarian tissue preservation in cancer patients is live birth, which can take several years to achieve given that the patient has to be disease-free and healthy. Other endpoints, which are less time consuming, have been used frequently to evaluate the effectiveness of different vitrification methods for ovarian tissue. These endpoints include tissue morphology, follicle counts, protein markers for follicle health and function, as well as in vitro (tissue and isolated follicle culture) and in vivo follicle development (xenograft and autograft). The advantages and disadvantages of these endpoints are discussed here.
Tissue histology is the most common endpoint and provides an evaluation of the health of ovarian follicles and stroma based on morphology. Using tissue histology as an endpoint is simple and produces quick results. In addition, morphological change is often the first indicator of cryodamage such as abnormal cellular shrinkage and swelling resulting in lysed cells. The disadvantage of using tissue morphology as the sole endpoint is the lack of functional evaluation. Damage to intracellular organelles and intercellular contacts, which are important for follicle development and survival, can be triggered by cryoprotectant toxicity and the vitrification procedure without apparent histological changes.
Tissue morphology is often accompanied with follicle counts. Follicle counts provide an estimation of follicle numbers in fresh vs. vitrified tissues. However, one must take great caution in interpreting these results. In primate ovaries, the distribution of follicles is heterogeneous and follicular density can vary more than two orders of magnitude in cortical tissues within the same ovary [33]. Therefore, it is difficult, if not impossible, to compare numbers of follicles in different treatment groups. In addition, follicles that had been lysed would not be detected following counting, resulting in an overestimation of follicular survival. For these reasons, follicular quantification and tissue morphology, while providing a quick and general evaluation of tissue health, are much more useful when combined with analyses such as protein and functional endpoints in evaluation of vitrification protocols.
Protein markers are routinely used in examining tissue and follicle health prior to and following vitrification. Protein expression/localization is most frequently assessed using immunohistochemistry, and markers used are typically related to cellular growth/proliferation, apoptosis pathways, ovarian follicle-specific proteins, and vasculature. Classical markers of cellular proliferation include Ki-67 [2], proliferating cell nuclear antigen (PCNA; [27]), and phospho-histone H3 (PPH3; [37]). Apoptosis markers are often used to indicate follicle death/atresia and include active caspase-3 [44]; Fas, Fas ligand, Bcl-2, Bax, and p53 [14]; and TUNEL assay [21]. Follicle-specific markers are used to assess the health and developmental potential of the ovarian follicles, and these include growth differentiation factor 9 (GDF-9), anti-Müllerian hormone (AMH; [2]), activin, and phosphorylated Smad 2 (p-Smad 2; [17]). Both GDF-9 and AMH are members of the TGFβ family. GDF-9, expressed in the cytoplasm of the oocyte, plays an important role during early folliculogenesis [28]. AMH is produced by granulosa cells of follicles from the primary to antral stage with its peak production in the small antral follicles [41]. Activin has been shown to be important for granulosa cell proliferation and follicle development, while Smad 2 is a downstream target of activin and is phosphorylated upon the activation of activin [12]. Finally, markers of vascularization are often used to assess the potential of the ovarian stroma to re-vascularize and support follicle survival and growth after transplantation. These markers include vascular endothelial growth factor (VEGF; [9]), a potent angiogenic factor that promotes growth of vascular endothelial cells, as well as CD31, also known as platelet endothelial cell adhesion molecule-1 (PECAM-1), an endothelial cell marker [21]. Endpoints such as tissue morphology and protein expression can give a rapid evaluation of the tested vitrification method; however, these endpoints do not reflect true ovarian function.
Other relatively short-term functional assays can be performed and are important for evaluating vitrification methods. Bromodeoxyuridine (BrdU) is incorporated into newly synthesized deoxyribonucleic acid (DNA) during the S phase of mitosis in dividing cells and can be used for the evaluation of tissue viability post-warming in culture. Vitrified ovarian tissues are cultured with BrdU for 48 h at 37 °C in 5 % CO2 and subsequently fixed and immunostained for BrdU [20]. BrdU incorporation is mainly observed in granulosa cells of preantral follicles as well as stromal cells. BrdU incorporation may be a more accurate reflection of post-thaw viability as well as the ability of cells to recover from cryopreservation procedures.
Individual secondary follicles isolated from vitrified tissue have been encapsulated in alginate and cultured to evaluate follicle survival, growth, and ability to form an antrum and produce hormones (i.e., estradiol, progesterone, and AMH) as well as generate mature oocytes and live births (in rodent models only) [10, 38]. Vitrified ovarian tissue pieces have also been cultured in vitro; however, the current tissue culture system is still suboptimal and does not support long-term tissue viability; increased atretic follicles are observed in pieces of ovarian cortex cultured for longer 5 days [43]. Nevertheless, in vitro culture of ovarian cortical tissue provides insights into tissue morphology as well as short-term functional endpoints such as hormone production [15], the ability of follicles to grow [38], and expression of protein markers mentioned above [32].
Another option for examining the function of vitrified ovarian tissue is xenotransplantation. A recent review by Dittrich and colleagues [4] summarizes studies wherein prepubertal or adult human ovarian tissue cryopreserved by slow-rate freezing was transplanted into various sites (kidney capsule, intra-abdominal pockets, subcutaneous spaces, intramuscular pockets) of immunocompromised mice with resultant recovery of ovarian function and, in a few cases, development of antral follicles from which metaphase II ooyctes were recovered. Xenotransplantation of cryopreserved human ovarian tissue provides several advances over other in vitro functional assays such as the ability to evaluate re-vascularization of the ovarian graft, as well as long-term (months) in vivo support of follicular development to the antral stage. However, daily injections of human follicle-stimulating hormone are required to support follicle survival and growth. While an additional and critical application of xenotransplantation of human ovarian tissue is to assess the risk of reintroducing malignant cells [4], the concept of maturing human oocytes within another species is not yet clinically acceptable to be used as a means of fertility restoration.
Autotransplantation of vitrified ovarian tissue can be performed in the nonhuman primate model and can provide crucial information regarding the viability and function of vitrified ovarian tissue. Several groups have used nonhuman primates to evaluate vitrification methods for ovarian tissue and these studies are discussed in the section below.
Nonhuman Primate Models of Ovarian Tissue Vitrification
While valuable, studies using human ovarian tissues suffer from the limited availability of human ovarian tissue for research. As a result, these studies often use pooled samples from different patients of various ages and reproductive backgrounds, resulting in a lack of systematic comparison. The nonhuman primate is an ideal animal model for obtaining ovarian tissue for vitrification studies, due to the vast similarities in reproductive physiology and anatomy, including ovarian structure and function, between nonhuman primates and women. In addition to our laboratory, two other groups have utilized the nonhuman primate model in efforts to optimize ovarian tissue vitrification techniques; the current results to date are discussed here.
Suzuki and colleagues [11] systemically examined two different vitrification solutions (VSED, ethylene glycol, dimethyl sulfoxide, and sucrose, vs. VSEGP, ethylene glycol, polyvinylpyrrolidone [PVP], serum supplement substitute [SSS], and sucrose) and three different equilibration times (5, 10, and 20 min) on the morphology of cynomolgus macaque ovarian tissue post-thaw. In this study, the authors utilized an open system that involved direct plunging of the tissue into liquid nitrogen. While resulting in a high cooling rate, direct contact between the tissue and liquid nitrogen poses a safety risk for possible contamination from direct contact of tissue with liquid nitrogen as well as cross contamination between specimens during storage [3]. The VSEGP vitrification solution preserved a higher proportion of morphologically normal follicles compared to VSED. Transmission electron microscopy of vitrified-thawed ovarian tissue revealed that vitrification caused vacuolated mitochondria, an elevated surface ratio of lysosomes per oocyte cytoplasm, as well as collapsed collagen bundles between follicles and stromal cells. However, of the two vitrification solutions tested, VSED caused increased cryodamage compared to VSEGP. In addition, a shorter exposure time (5 min) using the VSEGP vitrification solution preserved follicle morphology, normality of mitochondria, and lysosomes in preantral follicles, compared to longer exposure times (10 and 20 min). Using the optimal vitrification solution and exposure time combination, this group adapted a closed system (0.5 cc straws) for tissue safety and assessed long-term function of vitrified ovarian tissue following heterotopic transplantation in cynomolgus macaques [35]. The heterotopic sites included retroperitoneal iliac fossa, omentum, uterine serosa, and mesosalpinx. Recovery of ovarian cyclicity, based on circulating estradiol and progesterone levels, was confirmed in three of four monkeys between 78 and 207 days posttransplantation and was maintained for as long as 716 days. In animals with confirmed ovarian cycles, ovarian stimulation with exogenous gonadotropin treatment was performed, and oocytes were collected 40 h after human chorionic gonadotropin (hCG) administration. This was the first study in primates wherein oocytes (n = 9 total) were collected from preovulatory follicles derived from vitrified ovarian tissue transplanted to non-ovarian sties. Some of the oocytes underwent fertilization with subsequent early embryonic development (8- to 16-cell stages) in vitro, establishing the potential of ovarian tissue vitrification to yield healthy gametes and embryos. The authors also utilized contrast-enhanced computed tomography to monitor reestablishment of vascularization and blood flow to the ovarian grafts and found abundant vasculature in the greater omentum, suggesting this as an optimal site for heterotopic transplantation of vitrified-thawed ovarian tissue.
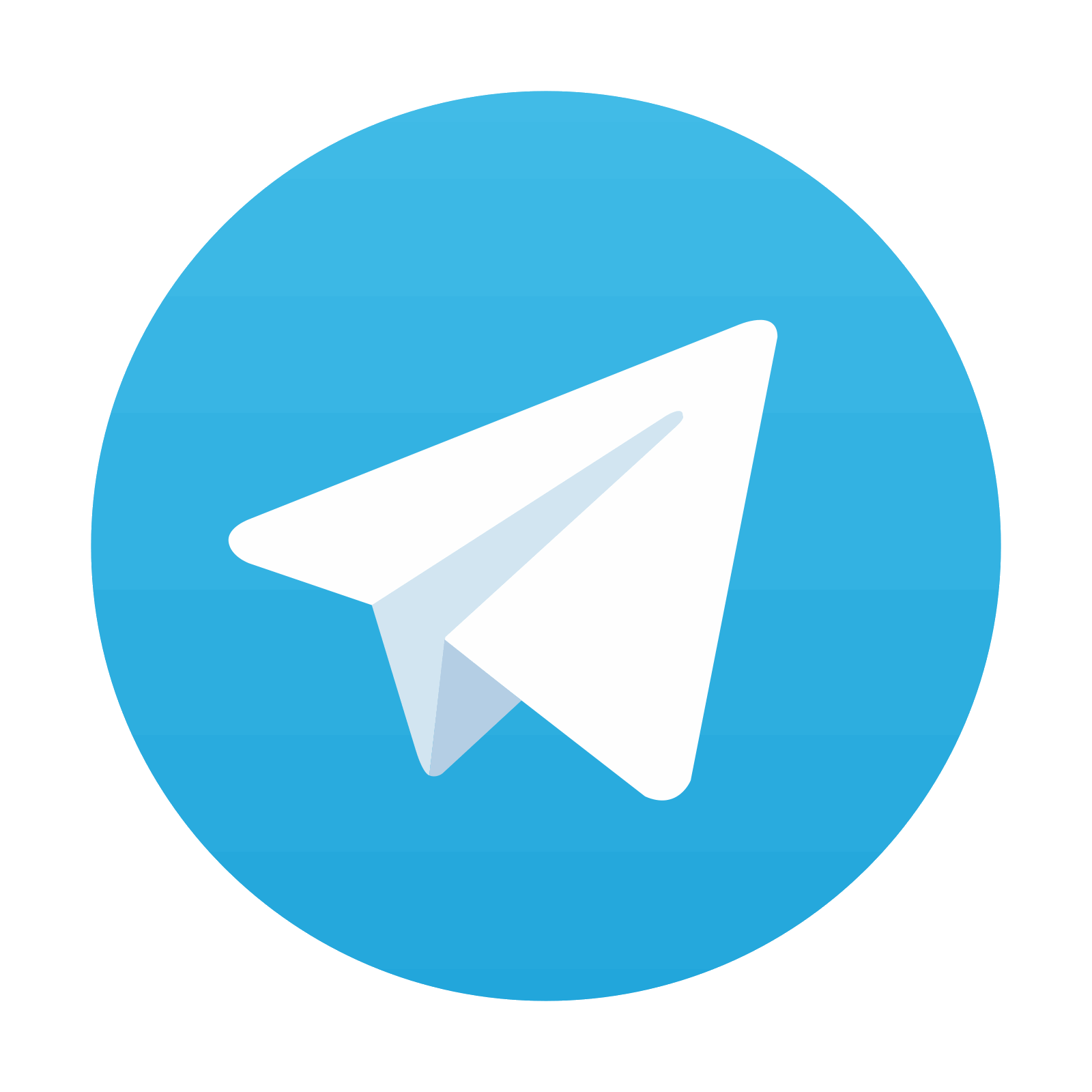
Stay updated, free articles. Join our Telegram channel
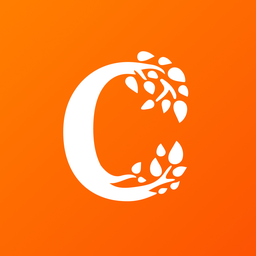
Full access? Get Clinical Tree
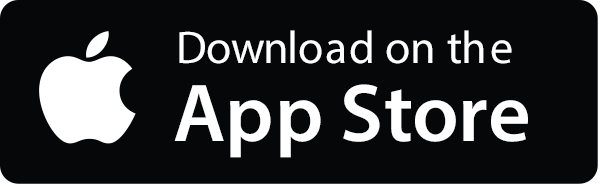
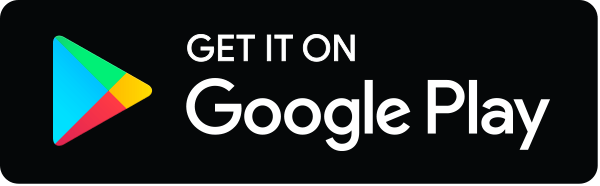