Fig. 8.1
Summary of signal transduction pathways operating downstream of vascular endothelial growth factor-A (VEGFA) in endothelial cells of relevance for vascular permeability in vivo . See text for details. Figure is based on Claesson-Welsh and Welsh [7]
In vivo studies of vascular permeability have implicated the participation of Src family kinases in this process. Experiments using Src kinase-deficient mice revealed reduced VEGFA-induced vascular permeability [27] and subsequently this was found to depend on phosphorylation of VE-cadherin [28] that destabilized adherens junctions. Consequently, tissue edema was reduced and recovery after myocardial infarction improved in the absence of Src activity.
Axl is another tyrosine kinase that contributes to VEGFA-stimulated vascular permeability in vivo [46]. Axl is downstream of TSAd/Src and is thought to regulate vascular permeability by activation of PI3K.
In vivo studies using endothelial-specific FAK gene inactivation have demonstrated the requirement of FAK for VEGFA-induced vascular permeability [48, 49].
Studies directly addressing the role of Rac1 in vascular permeability in vivo are sparse since mice deficient in endothelial Rac1 die in utero due to vascular abnormalities [82]. However, the absence of Rac1 activity in endothelial cells will diminish endothelial cell-driven vasodilation [83]. This effect may, in part, be a consequence of reduced eNOS activation, leading to less blood flow after induced hind-limb ischemia. However, increased endothelial cell Rac1 activity may under certain conditions give less vascular leakage, suggesting multiple modes of action of this signaling component in the vascular permeability response [60].
Absence of the p110γ isoform of PI3K causes diminished vascular permeability in response to VEGFA [84]. On the other hand, inactivation of class 1A PI3K activity leads to increased dye leakage in the neovasculature, suggesting a complex mode of action of PI3K in regulation of this process [85]. Increased activity of the PI3K downstream signaling intermediate Akt in endothelial cells causes chronically increased vascular permeability , with edema as a consequence [68]. This effect is at least partly due to vessel dilation and stimulation of eNOS. Conflicting results have been obtained on the effects of Akt deficiency. Whereas one report described reduced VEGFA-induced vascular permeability [86] as a consequence of Akt1 deficiency, another study noted an increased response [87]. The deficient VEGFA-stimulated vascular permeability was attributed to weak activation of eNOS, with decreased production of NO [86].
Shb is essential for VEGFA-stimulated vascular permeability in vivo [79]. The dissociation of VE-cadherin from adherens junctions that occurs in vivo in response to VEGFA fails as a consequence of Shb deficiency [26], providing a mechanistic explanation for the impaired vascular permeability response. Vascular permeability and blood flow in experimentally-induced muscle ischemia are reduced in the absence of Shb [26], suggesting a contribution of Shb and vascular permeability for recovery in this situation.
TSAd is required for VEGFA-induced vascular permeability [37, 38], both in the skin and the trachea. This effect is explained by impaired VEGFA-induced disassembly of adherens junctions [37]. TSAd signaling appears not to have a major effect on eNOS activity, and histamine-induced vascular permeability was unaffected by the absence of TSAd [37].
No data directly implicating IQGAP1 in the VEGFA-induced vascular permeability response are at present available, although IQGAP1 was shown to be required for macrophage infiltration into damaged muscle tissue during post-ischemic recovery [88].
GAB1 is necessary for post-ischemic recovery, VEGFA-induced angiogenesis and vascular permeability in vivo [71]. Although GAB1 is normally thought to mediate PI3K activation, the in vivo response of the knockout primarily depends on diminished activation of protein kinase A, leading to reduced activation of eNOS [71].
Several of the signal transduction responses downstream of VEGFR-2 ultimately cause activation of eNOS, as described above. This is an important effector since absence of eNOS will abolish the vascular permeability response [89]. As mentioned above, the mechanisms behind the permeability response of NO are poorly understood but may involve increased vasodilation and direct disruption of adherens junctions.
8.5 Modulation of VEGFA-Induced Vascular Permeability by Other Factors
Angiopoietin-1 is an angiogenic factor, operating via its receptor Tie2, which is known to reduce VEGFA-stimulated vascular permeability [90], although the underlying mechanism remains unclear. One proposed model involves RhoA-dependent sequestration of Src onto mDia (diaphanous homolog), thus reducing the phosphorylation of S665 on VE-cadherin in an Rac-dependent manner [91]. However, the effects of angiopoietin-1 on Rac1 activity are contradictory since another study described increased Rac1 activity in response to this ligand, causing reduced VEGFA-stimulated vascular leakage [60]. Apparently, Rac1 may stimulate or inhibit vascular permeability, depending on the local conditions. Angiopoietin-1 may also inhibit VEGFA-induced vascular permeability via protein kinase C zeta-dependent phosphorylation of an inhibitory site on eNOS [92]. Tie2 and VE-PTP interact on the cell surface but downregulation of such complexes does not affect the VE-cadherin staining pattern [93]. However, VE-PTP-dependent dephosphorylation of VEGFR2 requires angiopoietin-1-activated Tie2 [76], suggesting a VE-PTP-dependent antagonism between VEGFA and angiopoietin-1 regulating junction permeability. In addition, angiopoietin-1 decreases the phosphorylation of VE-cadherin at Y658, presumably via the action of VE-PTP, since VE-cadherin phosphorylation was elevated in VE-PTP-deficient cells.
The phospholipid sphingosine-1-phosphate is an extracellular ligand operating via G-protein-coupled receptors that influences the vasculature [94]. Sphingosine-1-phosphate may stimulate VEGFR2, resulting in Akt and eNOS activation [95]. In vivo, sphingosine-1-phosphate reduces vascular leakage by increasing Rac1 activity in a manner similar to that of angiopoietin-1 [60]. Alternatively, sphingosine-1-phosphate may stabilize vessels by inhibition of VEGFR2 signaling and retention of VE-cadherin at junctions after VEGFA stimulation [96].
8.6 VEGFA and Leukocyte Extravasation
VEGFA has been demonstrated to recruit leukocytes from the circulation to tissues, using different in vivo models. When VEGFA overexpression was induced in selected organs in a transgenic system, bone marrow-derived mononuclear myeloid cells were recruited to these specific sites [97]. Furthermore, neutrophils to accumulated at the site of intramuscularly transplanted pancreatic islets in a VEGFA-dependent manner [98, 99]. The revascularization process of these transplanted islets was dependent on recruitment of a distinct circulating neutrophil (CXCR4hi, MMP-9hi) population [98, 99]. The relationship between VEGFA, neoangiogenesis, and neutrophil extravasation is illustrated in Fig. 8.2. VEGFA production in transplanted islets promotes the extravasation of a subset of neutrophils that participate in the revascularization process.

Fig. 8.2
Schematic view illustrating neutrophil extravasation in relation to local vascular endothelial growth factor-A (VEGFA) production after islet transplantation to the cremaster muscle. Different steps of the leukocyte extravasation process (leukocyte rolling following capture, adhesion, crawling, and extravasation) are depicted that sequentially result in leukocyte migration towards the site of VEGFA production, which, in this scenario, is the transplanted islet. Leukocyte capture/rolling requires P-selectin on the endothelial cells and the P-selectin glycoprotein ligand-1 (PSGL1) expressed on the leukocyte. Expression of the endothelial cell adhesion molecule ICAM-1 and leukocyte integrins probably participates in this process. Consequently, an enrichment of a subpopulation of neutrophils (CXCR4hi, MMP-9hi) that assist neoangiogenesis occurs in the tissue surrounding the islet. The mechanisms by which they exert their proangiogenic effect are not completely understood, although they are likely to involve release of matrix metalloproteinase (MMP)-9. Islets devoid of VEGFA production fail to induce this process.
How tissue-derived VEGFA exerts its effect on leukocytes in circulation is still not clear, but diverse mechanisms have been suggested and may act in concert, potentiating each other. Studies performed in vitro on human umbilical vein endothelial cells (HUVECs) or colonic microvasculature demonstrated upregulation of endothelial adhesion molecules (ICAM-1 and P-selectin) in response to VEGFA [100–102], while VEGFA-induced leukocyte adhesion was shown to be dependent on CD18 [103, 104]. The expression of VEGFR1 on monocytes [97, 105] implies direct effects of VEGFA on cells in circulation. Indeed, VEGF-induced adhesion and migration of isolated monocytes, neutrophils, and T cells have been demonstrated [100, 101, 103, 106–108]. VEGFA is also known to induce expression of other chemotactic agents such as stromal cell-derived factor-1 (SDF-1). Transgenic induction of VEGFA expression induced expression of SDF-1 by fibroblasts, which, in the tissue, positioned recruited leukocytes perivascularly relative to the angiogenic vessels [97]. A role for SDF-1 in mobilization of circulating leukocytes at hypoxic sites has been reported [109]. In addition, multiple tumor-derived chemoattractants, including VEGFA, recruit CD11b+ Gr1+ leukocytes by activating the PI3-kinase isoform p110γ, which results in activation of the integrin α4β1 on the leukocyte, and concomitant tumor invasion [110].
Leukocyte extravasation can occur transcellularly through the thin endothelial cells, or paracellularly through endothelial junctions [111–113]. The extravasation process is mechanistically not yet well-defined and the route of choice might depend on stimulus, type of leukocyte, and location of the vascular bed. The importance of the paracellular route was demonstrated in two genetic models where the endothelial junctions were kept sealed by maintaining VE-cadherin in a constitutively active state [24, 77]. These models revealed greatly impaired leukocyte extravasation in response to inflammatory stimuli at different sites, demonstrating that opening of endothelial junctions is essential for leukocyte recruitment out of the vasculature. Whether opening of endothelial junctions during leukocyte extravasation inevitably results in a permeability increase remains to be shown.
Edema formation and accumulation of tissue leukocytes are cardinal signs of inflammation and these occur concomitantly with increased vascular permeability. Intuitively, an association between these processes is commonly made but several studies prove them to be both spatially and temporally uncoupled [114, 115], and leukocyte extravasation can occur at sites distant from formed endothelial gaps [116–118]. The formation of endothelial cell domes during leukocyte diapedesis has been implicated as a mechanism for controlling vascular permeability and endothelial barrier integrity [119]. Whether this is true for VEGFA-induced leukocyte recruitment has not yet been experimentally established. Thus, increased permeability identified by the hitherto applied methods appears not to be a prerequisite for leukocyte extravasation during inflammatory conditions.
Adherent leukocytes can produce and release reactive oxygen species (ROS) that induce opening of the endothelial junctions [120, 121]. The contribution of ROS production in leukocyte extravasation remains to be defined, and leukocytes lacking the ability to produce ROS are efficient in emigrating out of the vasculature. A recent study shows that the proangiogenic VEGFA-recruited neutrophils possess specific characteristics compared with neutrophils recruited to an infectious inflammation, since tenfold higher levels of the proangiogenic MMP-9 were found in the VEGFA-recruited population [99]. MMP-9 promotes angiogenesis both directly by potently digesting the extracellular matrix and thereby allowing for growth of newly formed vessels, and indirectly by releasing matrix-bound VEGFA [122]. Whether the distinct cell populations recruited by VEGFA produce similar amounts of ROS or other permeability-inducing factors remains to be shown.
The documented parallel effects of VEGFA on opening of endothelial junctions and leukocyte recruitment do not necessary imply contribution of the former to the latter. However, it seems plausible that VEGFA-induced weakening of endothelial junctions would facilitate leukocyte extravasation.
8.7 VEGFA-Induced Vascular Permeability and Disease
8.7.1 Vascular Permeability in Tumors
The tumor vasculature shows numerous abnormalities, such as poor perfusion, high vessel turnover, increased vessel tortuosity, and vascular leakage [123]. Antiangiogenic treatment by VEGFA-blocking regimens inhibits or reverses, in many instances, the abnormal tumor vessel phenotype and causes vascular ‘normalization’ [123]. Such strategies commonly inhibit tumor growth, and VEGFA inhibition is at present an accepted clinical practice for treatment of tumors such as glioblastomas unresponsive to other treatments, metastatic colorectal cancers, metastatic renal cell cancer, some nonsmall cell lung cancers, hepatocellular tumors, and neuroendocrine tumors (www.cancer.gov/cancertopics/factsheet/Therapy/angiogenesis-inhibitors). The reason why this therapy has proven relatively successful has nevertheless not been fully resolved since it has been shown that the VEGFA-inhibited tumor vasculature displays improved vascular function [123]. A possible explanation for this dichotomy is that the VEGFA-blocking treatments, in addition to reducing tumor angiogenesis, simultaneously inhibit VEGFA-induced vascular permeability, and that the latter contributes to tumor growth and dissemination. The angiogenic factor angiopoietin-1 decreases vascular permeability in vivo [124]. When tumors were treated with an angiopoietin-2 inhibitor (L1-7(N)), tumor vessel ‘normalization’ was observed but this effect was reversed by inhibition of angiopoietin-1, suggesting that angiopoietin-1 can reduce tumor vascular permeability by antagonizing angiopoietin-2 [125]. A consequence of vascular permeability could be leukocyte extravasation, and it has been shown that many tumors expand in a manner dependent on infiltration of class II macrophages (M2) [126, 127], which confer a proangiogenic phenotype. Indeed, VEGFA has been shown to cause selective extravasation of proangiogenic leukocytes [99], and such an effect could promote tumor expansion.
8.7.2 Vascular Permeability in Retinal Disease
The vasculature in the eye is protected by the blood–retinal barrier (BRB), which is maintained by tight junctions between retinal capillary endothelial (RCE) cells on the one hand, and retinal pigment epithelial (RPE) cells on the other, which form the inner and outer BRB, respectively [128]. The tight junctions of RCE cells are formed by intercellular communications between RCE and glial cells [129]. Thereby, organization of the BRB resembles the blood–brain barrier. Loss of normal BRB function is a common feature of many retinal degenerative disorders that are leading causes of visual dysfunction. Such diseases include age-related macular degeneration, diabetic retinopathy, and retinal vein occlusions [130] (see Chap. 12 by Hammes). Patients with age-related macular degeneration present focal ischemia of the outer retina, which induces VEGFA production and angiogenesis , resulting in hyperpermeable vessels and inflammation. Prolonged elevation of blood sugar levels in diabetic patients causes endothelial apoptosis, basement membrane thickening, and pericyte loss, accompanied by increased VEGFA synthesis and vascular permeability. Retinal vein occlusions can be attributed to hemodynamic disturbances, such as increased coagulation and impaired flow properties, resulting in ischemia and increased VEGFA synthesis (see Stewart [130] for details). Therefore, common aspects of many vascular eye diseases are ischemia, increased VEGFA production, and excess vascular permeability [131]. The excess permeability has been attributed to both the overstimulated, abnormal vasculature and to changes in the phoshorylation of tight junction proteins such as occludin and zona occludens protein-1 (ZO1) [132]. Bevacizumab, a VEGFA neutralizing antibody or Lucentis, a VEGFA neutralizing Fab2 fragment, administered by intravitreal injection, have been used successfully for the treatment of ocular diseases, resulting in preservation and even gain of visual acuity.
Figure 8.3 summarizes VEGFA-induced vascular permeability in the context of pathophysiological processes, and suggests possible modes of pharmacological intervention.

Fig. 8.3
Vascular endothelial growth factor-A (VEGFA)-induced vascular permeability in the context of disease. Potential sites of pharmacological intervention include VEGFA/VEGF receptor-2 (VEGFR2) antagonists/inhibitors, angiopoietin-1, angiopoietin-2 inhibitor, sphingosine-1 phosphate, and intracellular VEGFR2 signaling. See texts for details
8.8 Conclusions
Numerous signaling mechanisms participate in the response, conferring vascular permeability in response to VEGFA and significant redundancy exists between the various pathways. Presumably, precise control of the characteristics and degree of stimulation of vascular permeability can be achieved by differentially orchestrating the individual signaling pathways. In addition, this pleiotropy ascertains that the permeability response will not be suppressed in case one signaling scheme fails. Taken together, this indicates the importance of vascular permeability for the tissue repair response to ischemia. It is still uncertain what the precise beneficial effects of vascular permeability for the repair processes are, but they could involve extravasation of both plasma proteins and leukocytes . A factor that complicates specific elucidation of the beneficial contribution of vascular permeability to tissue restoration is that most signal transduction pathways of importance in this context are also essential for tissue angiogenesis [7], making delineation of the relative importance of vascular permeability versus angiogenesis challenging. In fact, one may speculate that these are two inseparable aspects of the angiogenic repair process. Inhibition of tumor angiogenesis by VEGFA-blocking regimens has been assumed to be the main targets of such treatments, but it is conceivable that inhibition of VEGFA-induced vascular permeability may be an equally important component.
Acknowledgments
The study was supported by grants from Diabetes Wellness Sweden (MP), the Novo Nordisk foundation (MP), the Swedish Cancer Fund (MW, LCW), the Swedish Research Council (MP, MW, LCW), the Swedish Diabetes Fund (MP, MW), the Ragnar Söderberg foundation (MP), the Knut and Alice Wallenberg Foundation (MP, LCW), and the Family Ernfors Fund (MP, MW).
References
1.
Ferrara N. Vascular endothelial growth factor: basic science and clinical progress. Endocr Rev. 2004;25(4):581–611.PubMed
2.
Keck PJ, Hauser SD, Krivi G, Sanzo K, Warren T, Feder J, et al. Vascular permeability factor, an endothelial cell mitogen related to PDGF. Science. 1989;246(4935):1309–12.PubMed
3.
Senger DR, Perruzzi CA, Feder J, Dvorak HF. A highly conserved vascular permeability factor secreted by a variety of human and rodent tumor cell lines. Cancer Res. 1986;46(11):5629–32.PubMed
4.
McDonald DM, Thurston G, Baluk P. Endothelial gaps as sites for plasma leakage in inflammation. Microcirculation. 1999;6(1):7–22.PubMed
5.
Dvorak HF. Angiogenesis: update 2005. J Thromb Haemost. 2005;3(8):1835–42.PubMed
6.
Sprague AH, Khalil RA. Inflammatory cytokines in vascular dysfunction and vascular disease. Biochem Pharmacol. 2009;78(6):539–52.PubMedCentralPubMed
7.
Claesson-Welsh L, Welsh M. VEGFA and tumour angiogenesis. J Intern Med. 2013;273:114–27.PubMed
8.
Maurer M, Bader M, Bas M, Bossi F, Cicardi M, Cugno M, et al. New topics in bradykinin research. Allergy. 2011;66(11):1397–406.PubMed
9.
Kumar P, Shen Q, Pivetti CD, Lee ES, Wu MH, Yuan SY. Molecular mechanisms of endothelial hyperpermeability: implications in inflammation. Expert Rev Mol Med. 2009;11:e19..PubMedCentralPubMed
10.
Nagy JA, Benjamin L, Zeng H, Dvorak AM, Dvorak HF. Vascular permeability, vascular hyperpermeability and angiogenesis. Angiogenesis. 2008;11(2):109–19.PubMedCentralPubMed
11.
Dvorak HF, Quay SC, Orenstein NS, Dvorak AM, Hahn P, Bitzer AM, et al. Tumor shedding and coagulation. Science. 1981;212(4497):923–4.PubMed
12.
Nagy JA, Dvorak AM, Dvorak HF. Vascular hyperpermeability, angiogenesis, and stroma generation. Cold Spring Harb Perspect Med. 2012;2(2):a006544.PubMedCentralPubMed
13.
Majno G, Shea SM, Leventhal M. Endothelial contraction induced by histamine-type mediators: an electron microscopic study. J Cell Biol. 1969;42(3):647–72.PubMedCentralPubMed
14.
Kohn S, Nagy JA, Dvorak HF, Dvorak AM. Pathways of macromolecular tracer transport across venules and small veins. Structural basis for the hyperpermeability of tumor blood vessels. Lab Invest. 1992;67(5):596–607.PubMed
15.
Roberts WG, Palade GE. Increased microvascular permeability and endothelial fenestration induced by vascular endothelial growth factor. J Cell Sci. 1995; 108(Pt 6):2369–79.PubMed
16.
Simionescu M, Simionescu N, Palade GE. Differentiated microdomains on the luminal surface of capillary endothelium: distribution of lectin receptors. J Cell Biol. 1982;94(2):406–13.PubMed
17.
Schubert W, Frank PG, Woodman SE, Hyogo H, Cohen DE, Chow CW, et al. Microvascular hyperpermeability in caveolin-1 (-/-) knock-out mice. Treatment with a specific nitric-oxide synthase inhibitor, L-NAME, restores normal microvascular permeability in Cav-1 null mice. J Biol Chem. 2002;277(42):40091–8.PubMed
18.
Adamson RH, Zeng M, Adamson GN, Lenz JF, Curry FE. PAF- and bradykinin-induced hyperpermeability of rat venules is independent of actin-myosin contraction. Am J Physiol Heart Circ Physiol. 2003;285(1):H406–17.PubMed
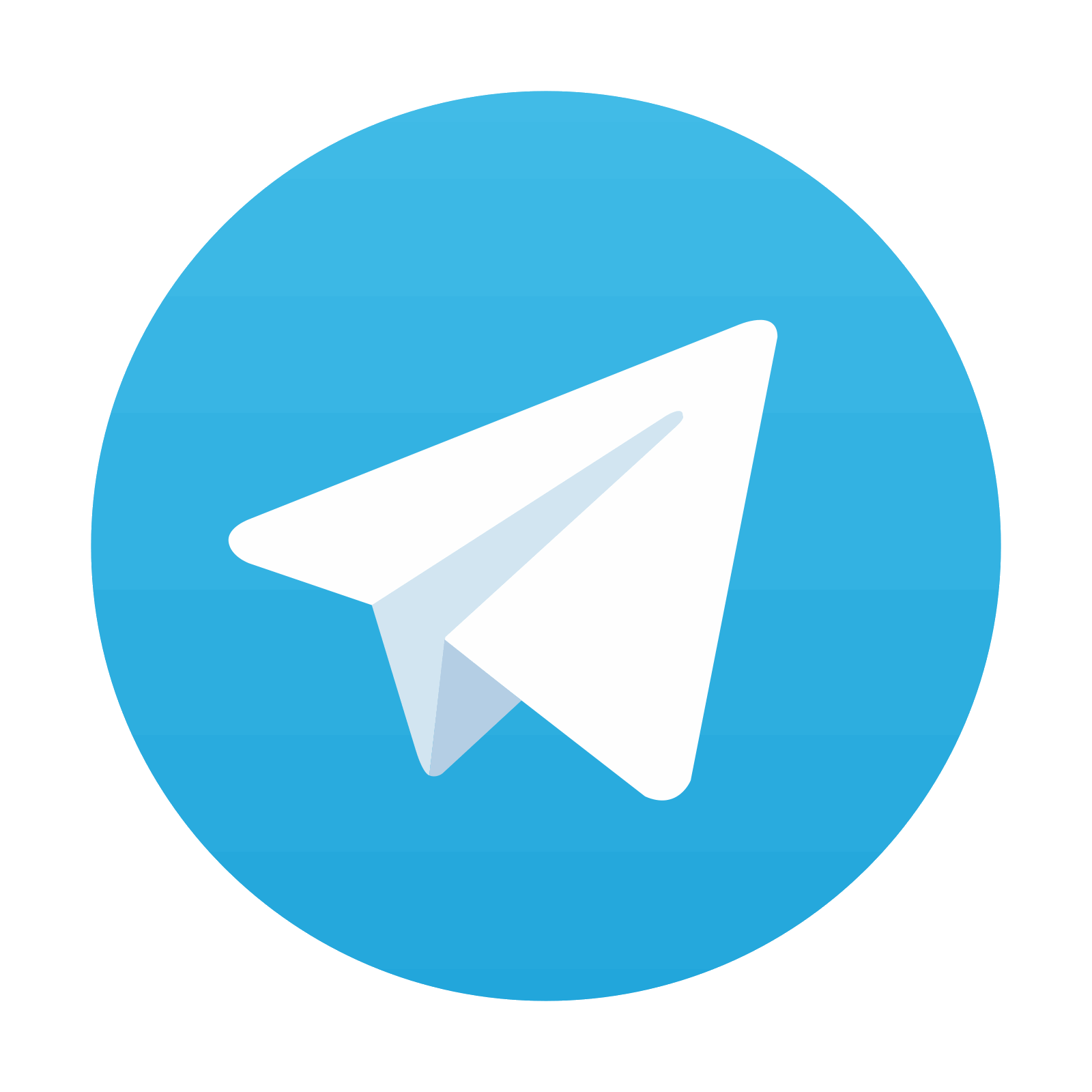
Stay updated, free articles. Join our Telegram channel
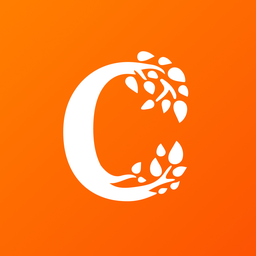
Full access? Get Clinical Tree
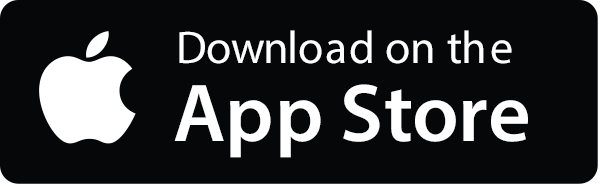
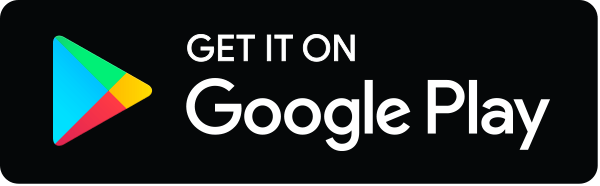