Fig. 2.1
Arterial and venous precursor cell migration. a At 12 hpf, angioblasts are located within the anterior and posterior lateral plate mesoderm (LPM) in two bilateral stripes. Presumably, even at this early stage, the medial angioblast population consists of arterial precursor cells (red), while the lateral population consists of venous precursor cells (blue). b At approximately 14 hpf, the precursor cells located at more medial positions within the posterior LPM start to migrate to the midline. At the same time, some arterial cells of the posterior LPM start to migrate anteriorly, whereas arterial cells from the anterior LPM start to migrate posteriorly. c At 16 hpf, arterial precursor cells will form the first axial vessel, the dorsal aorta. Cells within the more lateral located stripes of the posterior LPM start to migrate to the midline. In addition, some of these cells will migrate anteriorly, whereas in the anterior LPM some venous progenitor cells start to migrate posteriorly. d At 20 hpf, the venous precursor cells have migrated to the midline and form the posterior cardinal vein (PCV). The migration of arterial cells in the anterior region results in formation of the lateral dorsal aortae. e At 24 hpf, venous precursor cells in the anterior region have migrated to form the Primordial hindbrain channel (PHBC). Green indicates somites, purple indicates notochord and hypochord, yellow indicates embryonic tissue
How about the movement of these angioblasts from the posterior LPM to the midline? As mentioned above, it has been reported a number of times that first the medial, then the lateral, angioblast population migrates to the midline. Both migration waves occur in an anterior to posterior manner. The use of a transgenic line that expresses the photo-convertible fluorophore kaede from the etv2 promoter has enabled elegant studies which demonstrated that the medial angioblasts give rise to the DA, and that lateral angioblasts constitute the PCV [39] (Fig. 2.1). Furthermore, these studies support a model where angioblasts move directly to a more dorsal location and form the DA, while lateral angioblasts migrate directly to a more ventral position and form the PCV. This is in contrast to a study by Herbert et al. [26], who suggested a ventral sprouting mechanism, during which angioblasts from the DA contribute directly to the PCV [26]. Whether this discrepancy is possible due to imaging in different regions of the embryo needs to be resolved.
As a consequence of early specification events and a highly orchestrated (both in time and space) array of cell movements, two cords of angioblasts/endothelial cells align along the embryonic axis. These cells establish cell–cell junctional complexes among each other, express markers of apical and basal polarity, and eventually form a luminized DA and PCV, even before the onset of circulation (reviewed by Schuermann et al. [65]).
2.2 Molecular Cues During Vasculogenesis
There is a plethora of genes and factors that have been connected to the genetic control of vasculogenesis and angiogenesis, but only a few for which zebrafish mutants are available and for which we fully understand the mechanistic implications. One of the first cardiovascular mutants described presents with a near-complete failure to specify blood and endothelial lineages, and these cloche mutants have been very instructive to understand many aspects of early vascular development [72] . It has been suggested that mutations in the lycat gene, encoding an acyl transferase, are causative for the phenotype [86]. The cloche mutant phenotype can be rescued via forced expression of the ETS1-related protein, placing this key transcription factor downstream of cloche [74]. As mentioned above, the ETS-domain transcription factor Etv2/Etsrp/ER71 is one of the earliest markers specifically expressed in angioblasts, and Etsrp is required for the expression of vegfr2/kdrl in early development. In etsrp zebrafish morphants, angioblasts are unable to differentiate, migrate, or form functional axial vessels. Overexpression of etsrp causes the induction of vascular endothelial markers in several cell types. Etsrp is thus a key regulator in the induction of vascular endothelial fate in early development [58, 74].
Overexpression studies showed that vascular endothelial growth factor A (vegf-a) and Sonic Hedgehog (Shh) are involved in the localization of the medial and lateral angioblasts to the midline, with high levels of vegf-a or shh resulting in a random distribution of medial and lateral angioblasts at the midline [39]. Similarly, vegf-a morphants showed a single vessel consisting of lateral and medial angioblasts [39], suggesting that Vegf and Shh are critical factors for arteriovenous specification at the time point of angioblast positioning at the midline. In zebrafish , shh is expressed in the notochord and floorplate [16] , whereas vegf-a is expressed in the somites [49]. It is hypothesized that the medially located angioblasts receive a higher concentration of Shh and Vegf signaling than the angioblasts localized at the lateral positions [39]. This difference in concentration could induce the distinct pathways for arterial or venous specification. This model is intriguingly simple but important questions remain. Are these morphogens (Shh, Vegf) sufficient to both serve as chemoattractants for cell migration and for specifying arterial and venous cell fates? Or is there a stochastic initiation of some angioblasts in the LPM to migrate medially in a first wave, and these ‘front runners’ inhibit arterial fates in the trailing cells of the second wave? A thorough fate mapping of migration events might clarify some of these issues; if all angioblasts are equally naïve before the onset of migration, then fate mapping should reveal that the cells located most medially in the LPM (and which should therefore perceive the highest levels of Vegf and Shh) should invariably end up in the aorta. However, should more medially located cells be overtaken by more distally located cells, this would argue that the cells might not be naïve .
While the axial vasculature is defective in vegf-deficient embryos and shh mutants, the anterior vasculature is unaffected, suggesting a different mechanism of vessel formation in the anterior LPM of the zebrafish . Time-lapse analysis showed that the anterior part of the DA, the lateral DA (LDA) develops in a different manner. At 14 hpf, a subset of angioblasts localized at the anterior LPM migrate posteriorly, while a subset of angioblasts from the posterior LPM start to migrate anteriorly. These cell populations migrate towards each other, eventually connecting and forming the LDA. This process of angioblast migration is suggested to be mediated by the chemokine Cxcr4 [70]. The expression of cxcr4 is restricted to the angioblasts that will form the anterior-most LDA. Knockdown of cxcr4 resulted in inappropriate fusion of anterior and posterior angioblasts, and consequently formed a disrupted LDA [70]. At approximately 17 hpf, the same process of migration happens for venous angioblasts that form the largest anterior vein in the head, the primordial hindbrain channel (PHBC) [7, 70] (Fig. 2.1 and 2.2a, b).

Fig. 2.2
Transgenic zebrafish vasculature. a Overview of kdrl:mCherry;flt4:mCit zebrafish embryo at 2 days post-fertilization (dpf), with arteries depicted in red and veins depicted in yellow. b Enlarged head region of (a), including the heart (H), lateral dorsal aorta (LDA), and the posterior hindbrain sinus primordial hindbrain channel (PHBC). c Trunk region of flt1:tdTomato;flt4:mCit embryo at 3 dpf, with arteries depicted in red and veins depicted in green, including the arterial intersegmental vessel (aISV), venous intersegmental vessel (vISV), dorsal aorta (DA), and posterior cardinal vein (PCV)
After the initiation of vasculogenesis , angiogenesis will further remodel the vascular network. Angiogenesis is the formation of blood vessels from pre-existing blood vessels by sprouting and remodeling of endothelial cells . In the zebrafish trunk, endothelial cells start to sprout dorsally from the DA at the somite boundaries and form the intersegmental vessels (ISVs). This primary sprouting results in ISVs that connect at the dorsal site to form the dorsal longitudinal anastomotic vessel (DLAV). After establishing this arterial network, secondary sprouting will occur in which endothelial cells sprout dorsally from the PCV [32]. These sprouts can connect to the ISVs and remodel the arterial ISV into a venous ISV. Statistically, only every second sprout becomes a venous ISV. The other endothelial sprouts will migrate further to the horizontal myoseptum and constitute a population of parachordal lymphangioblasts, which then migrate either dorsally or ventrally and start to form the lymphatic vasculature [29] (reviewed by van Impel and Schulte-Merker [77, 78]) (Fig. 2.2c).
One of the prevalent questions concerning the above angiogenic processes is how venous sprouts make the decision on whether to connect to an intersegmental artery and to remodel it into a vein in the process, or whether not to do this and contribute to the pool of lymphatic precursor cells. One might presume that this process might involve Notch and Delta but, until now, no members of the Notch/Delta signaling pathway have been detected to be expressed in the PCV or in venous sprouts.
2.3 Molecular Regulation of Arterial-Venous Specification
2.3.1 EphrinB2/Eph Receptor B4
Two of the most widely referred to markers for arteriovenous specification are Ephrin-B2 (Efnb2) and Eph receptor B4 (EphB4), based on the finding that Efnb2 and the EphB4 receptor are differentially expressed in arteries versus veins, respectively [81]. The EphB4 receptor belongs to the receptor tyrosine kinase family and is the only Ephrin receptor that specifically binds to Efnb2, a membrane-bound ligand of the Ephrin ligand family [20] . The Ephrin ligands and Eph receptors are both transmembrane proteins, and signaling requires cell-to-cell contact, which can be bidirectional [5]. Eph receptors and their ligands are often, but not always, localized in the adjacent cell population [1, 19]. ‘Forward’ signaling starts with the binding of an Ephrin ligand to a receptor dimer. This leads to trans-phosphorylation of the intracellular domain of the receptor, and results in a conformational change that can activate the kinase domain. ‘Reverse’ signaling occurs when the conserved tyrosine residues of the cytoplasmic domain of the Ephrin ligand are phosphorylated upon contact with the Eph receptor ectodomain, or by an Eph receptor-independent mechanism. This causes the recruitment of an SH2 (Src-homology-2) domain-containing adaptor protein and SH3 binding partners (reviewed by Kullander and Klein [41]). The exact contribution of forward and/or reverse signaling in arteriovenous specification is still unclear.
In zebrafish , efnb2 expression is restricted to the arterial endothelial cells (ECs). Expression of efnb2 is initiated at approximately the 20-somite stage, when the angioblasts have migrated to the midline but circulation has not yet commenced. ephb4 receptor messenger RNA (mRNA) is also expressed in the vasculature but the expression is restricted to the venous ECs [87]. These clear expression patterns in arterial versus venous ECs make EphB4 and Efnb2 suitable markers for arterial and venous differentiation and, accordingly, their expression changes upon altered arteriovenous specification. For example, inhibition of Notch signaling results in a decrease of arterial fates, which can be appreciated by reduced expression of efnb2 in the DA [44]. Herbert et al. suggested that Efnb2a limits the ventral migration of arterial angioblasts, whereas Ephb4a promotes it, based on results obtained upon transplanting efnb2a or ephB4a morpholino (MO) donor cells into wild-type host embryos. In hosts that received efnb2a MO donor cells, the donor cells ectopically localized to the vein. In host embryos that contain ephb4 MO donor cells, fewer cells contribute to the vein compared with their controls, again suggesting a role for Efnb2 and EphB4 in arteriovenous specification [26]. This is largely consistent with other systems, and mice mutants for Efnb2 have been shown to present the same phenotype as mutants for EphB4, characterized by defective morphogenesis of the vasculature. Mutant vasculature suffers from a lack of distinct boundaries between the arteries and the veins, again stressing the importance of Efnb2 and EphB4 in arteriovenous specification [20] .
2.3.2 Vascular Endothelial Growth Factor and Sonic Hedgehog
In zebrafish , as in all other non-eutherian vertebrates, four VEGF receptors are present, namely vegfr1 (flt1), vegfr2 (flk1/kdr), vegfr3 (flt4), and vegfr4 (kdrl) [6, 14, 75]. The expression pattern of these receptors has been examined in detail [6, 50, 68], and transgenic reporter lines exist for most of them. Neuropilins, non-tyrosine kinase transmembrane molecules, have been shown to be needed for VEGF signaling in other systems, but in zebrafish, morpholino-based data [47, 51] are not entirely consistent with recently provided mutant data [40], and generating mutant lines for the duplicated nrp1a/b and nrp2a/b genes is required to shed light on a requirement for these co-receptors.
Morpholino-mediated knockdown of vegf-Aa was reported to result in deficiency of ISV sprouting, with no major deficiencies in the DA or PCV [53]. However, analyzing the dependence of vasculogenesis on Vegf-A is confounded by the duplication of zebrafish vegf-A genes [3], and by maternal expression of the respective mRNAs. Stable mutant lines or maternal zygotic mutants have not been generated, precluding a final assessment on the role of Vegf-A during the early stages of vasculogenesis; current evidence based on double knockdowns suggests diverged functions of Vegf-Aa and Vegf-Ab, but no major effects on vasculogenesis [3]. Moreoever, mutants for vegfr4/kdrl [23] and vegfr3/flt4 [30] have been reported and clearly demonstrate that zygotic expression of these receptors is not essential for vasculogenesis to occur; mutants in either gene have an apparently normal DA and PCV. These genes have distinct functions at the later stages of vascular development , during arterial and venous ISV sprouting, but their role during earlier stages of vasculogenesis remains somewhat enigmatic; more than one VEGF receptor might be required to be mutated in order for a phenotype to be appreciated.
A key regulator in the early steps of angioblast migration, and in later events of arterial and venous specification, has been suggested in Shh, a member of the Hedgehog family which can act as a ligand for the transmembrane receptors Patched and Smoothened [45]. Smoothened mutants, which are devoid of Shh signaling, show comparatively normal angioblast migration [85], and the receptors for Shh, the duplicated patched genes, appear not to be expressed within the posterior LPM [48]. Shh regulates expression of semaphorin 3a1, which has been shown to have an effect on angioblast migration [69]. In addition, Shh signaling from the midline is essential for normal vegf-A expression in the medial aspects of the somites [45]. While more work needs to be carried out to clearly carve out the role for Shh and Vegf-A during the very first events of vasculogenesis , the requirement for both genes in the later steps of arteriovenous specification is better understood. Shh mutants show strongly reduced arterial marker gene expression, but this phenotype can be partially overcome by forced expression of vegf-A mRNA. Furthermore, overexpression of shh results in ectopic expression of arterial markers in venous ECs, again suggesting a specific role of Shh in arterial specification [21]. Interestingly, Shh has been demonstrated to positively influence the expression of calcitonin receptor-like receptor-a (calcrla) [54], which ultimately results in vegf-A expression upstream of Notch. This is particularly significant in light of a recent finding by Wilkinson et al. [85], where Hedgehog signaling was found to induce somitic vegf-A expression independent of Calcrla, while Hedgehog can also signal through Calcrla to induce arterial differentiation in angioblasts independent of Vegf-A function. Hence, at least during the later stages, there is room for both signaling pathways in parallel, which in turn might help to guide our thinking about a possibly redundant function for Shh and Vegf-A during the first steps of vasculogenesis. Indeed, it has been suggested that neither pathway is absolutely required for angioblast migration, and that one pathway can compensate for (partial) loss of the other. However, the requirement at the later stages is supported by a number of observations, and a picture has emerged where VEGF signaling in presumptive arteries induces Plc-γ1. Zebrafish mutants for plc-γ1 show a marked defect in the formation of arteries and strongly reduced expression of efnb2 [46]. plc-γ1 mutants cannot be rescued with vegf-A overexpression, suggesting Plc-γ1 to act downstream of VEGF receptor function in arterial signaling [44, 46].
2.3.3 Notch and Hey2
The zebrafish Notch family members consist of four Notch receptors (Notch1a, 1b, 2, and 3) and several Notch ligands (DeltaA-D, Dll4, Jagged1a, 1b, and 2) in zebrafish , which are all membrane-bound proteins. Upon binding of these ligands to the Notch transmembrane receptor, a series of proteolytic cleavages release the Notch intercellular domain (NICD) of the receptor into the cytoplasm, after which it translocates to the nucleus. The NICD can then bind to Suppressor of Hairless [Su(H)], which in turn can cause activation of several transcription factors, such as the basic helix-loop-helix (bHLH) proteins, Hairy/Enhancer of Split (Hes) and Hes-related proteins (Hey/HRT/HERP). The promoter regions of HRT genes have a binding site for Su(H) [36, 52]. The Notch signaling pathway has long been recognized as a key driver of arterial identity, and in recent years has been extensively evaluated in this respect, and also in its involvement during tip cell/stalk cell formation [59]. The latter aspect has been reviewed in detail elsewhere [84], therefore we will focus only on the arteriovenous specification role of Notch–Delta signaling.
The Notch–Delta signaling pathway appears to be restricted to the arterial endothelium in zebrafish [79], and both loss-of-function as well as gain-of-function studies of Notch family members revealed a disrupted vasculature, with loss of Notch signaling, such as in mindbomb mutant embryos, resulting in decreased arterial marker expression and arterial-venous shunts [44]. Similarly, mutants in hey2/grl, a factor required downstream of Notch signaling, display altered arterial gene expression and develop a distinct shunt phenotype at the level of the cranial vasculature [87]. Gain-of-function of Notch family members causes a reduction of venous fate [44]. There is a tight link between Vegf signaling function and Notch–Delta activity; Vegf-A can induce the expression of notch, and Notch can rescue the arterial specification defect in vegf-A knockdown studies, suggesting that Notch acts downstream of Vegf in arterial specification [44, 79]
One of the Notch target proteins is the hairy/enhancer-of-split-related bHLH family member Hey2. The zebrafish ortholog of the mammalian Hey2 gene is gridlock. Gridlock functions as a transcriptional repressor, and is already expressed in early development, in the angioblasts that are localized within the medial aspect of the LPM, whereafter gridlock expression continues to be restricted to arteries [87]. The loss of Gridlock function in early zebrafish development results in defective proliferation of angioblasts at the level of the LPM [10]. Later on, loss of gridlock results in a circulatory shortcut through a disrupted DA, with concomitant increase of the venous maker EphB4 and a decrease in the arterial marker efnb2 [87]. More specifically, the point of fusion of the LDA to the DA is affected, which represents a remarkably specific and locally restricted phenotype [83, 88] which has recently been shown to be mimicked by the sox7 and efnb2a/b mutants [27]. Furthermore, overexpression of gridlock causes suppression of venous markers [88]. Both in vitro and in vivo experiments showed that induction of Notch–ICD induces gridlock expression, again suggesting that Gridlock is acting downstream of Notch [52, 88]. Furthermore, inhibiting Hh or VEGF signaling results in loss of gridlock expression in the angioblasts that will form the DA, while stimulating vegf expression in gridlock morphants rescues the gridlock phenotype. These results show that gridlock functions downstream of the Hh–VEGF–Notch signaling pathway in arterial specification [57, 62]. As in other cases, the defect observable in mutants appears to be more pronounced in arteriovenous specification during angiogenesis and less during vasculogenesis .
2.3.4 SoxF Family Members
SRY-related high-mobility-group box (Sox) genes form a family of transcription factors involved in diverse developmental processes, including vascular development (reviewed by Chew and Gallow [9]). The Sox proteins contain two main domains—the high-mobility-group (HMG) domain, which can bind target DNA motifs, and the transactivation domain, which mediates the transcriptional response [4]. The Sox family is divided into several subfamilies, with the Sox-F family members comprised of the Sox7, Sox17, and Sox18 genes. Sox17 is involved in hematopoietic stem cell regulation and formation of endoderm [2, 11, 35, 37], but has also recently been shown to play a role in arterial specification in the mouse [12]. Sox7 and Sox18 have long been recognized as being involved in vascular development [8, 28, 55, 82]. The human syndrome hypotrichosis-lymphedema-telangiectasia (HLT) is linked to mutations in Sox18, with patients presenting disrupted blood and lymphatic vessels [31]. Mice with truncated Sox18 protein (‘Ragged’ mice) resemble HLT and display defects in blood and lymphatic vasculature development [15, 33, 56]. In mice, Sox18 starts to be expressed at approximately E9.0 in a subpopulation of venous endothelial cells, which induces Prox1 expression in these cells. Subsequently, these Prox1-expressing cells migrate away from the veins under the influence of VEGF-C and become lymphatic endothelial cells (LECs) which will later form the lymphatic vascular network [18, 24, 71] (see also Chap. 5). In contrast, zebrafish Prox1 and Sox18 are dispensable for lymphatic development, revealed by Prox1 and Sox18 mutants developing a lymphatic vasculature [77, 78].
In zebrafish, Sox18 and Sox7 appear to play redundant roles in vascular development. Sox7 and sox18 are expressed in the early pre-migratory angioblasts at the LPM, then in the migrating angioblast population and later in the specified vasculature. Double morpholino knockdown showed defective blood circulation, and the DA and PCV are fused together in the trunk of the embryo, which results in arteriovenous shunt formation at a relatively late stage of development. Venous markers are upregulated in the DA, whereas the arterial markers are downregulated, suggesting a role for Sox7/Sox18 in arteriovenous specification [28, 55]. This is further substantiated by the phenotype of sox7 mutants, which show phenotypes identical to gridlock and efnb2a/b mutants [27]. The exact mechanism of Sox7 and Sox18 function in arteriovenous regulation is not yet known; however, recent evidence showed that the enhancer for the Notch ligand Dll4 contains a binding site for SoxF factors. Both SoxF and RBPJ transcription factors can bind and regulate Dll4 enhancer activity, suggesting an important role for SoxF family members in regulating Dll4 activity and subsequent arteriovenous specification [64].
2.3.5 Fox and ETS
Forkhead (fox) transcription factors are helix-turn-helix proteins. Foxc transcription factors are expressed in the vasculature and Foxc-null mice die during embryonic development with severe vascular defects, including arteriovenous malformations and loss of arterial markers [42, 67]. Similarly, in zebrafish, combined knockdown of foxc1a and foxc1b results in severe disruption of the vascular system [13]. In vitro studies showed that VEGF signaling can induce the transcriptional activity of Foxc proteins [67]. Overexpression of foxc genes induces expression of arterial markers, such as notch1 and dll4 . Foxc can bind and activate the Dll4 promoter, suggesting that Foxc acts upstream of Notch signaling in arteriovenous specification [67]. Furthermore, Foxc2 can interact with the Su(H)/NICD complex to induce Hey2 promoter activity [25]. Foxc, together with the Ets factor Etsrp, bind to a FOX:ETS motif-inducing enhancer activation. This FOX:ETS domain is present in many endothelial-specific enhancers, suggesting the importance of the Foxc and Ets transcription factors in vascular development [13].
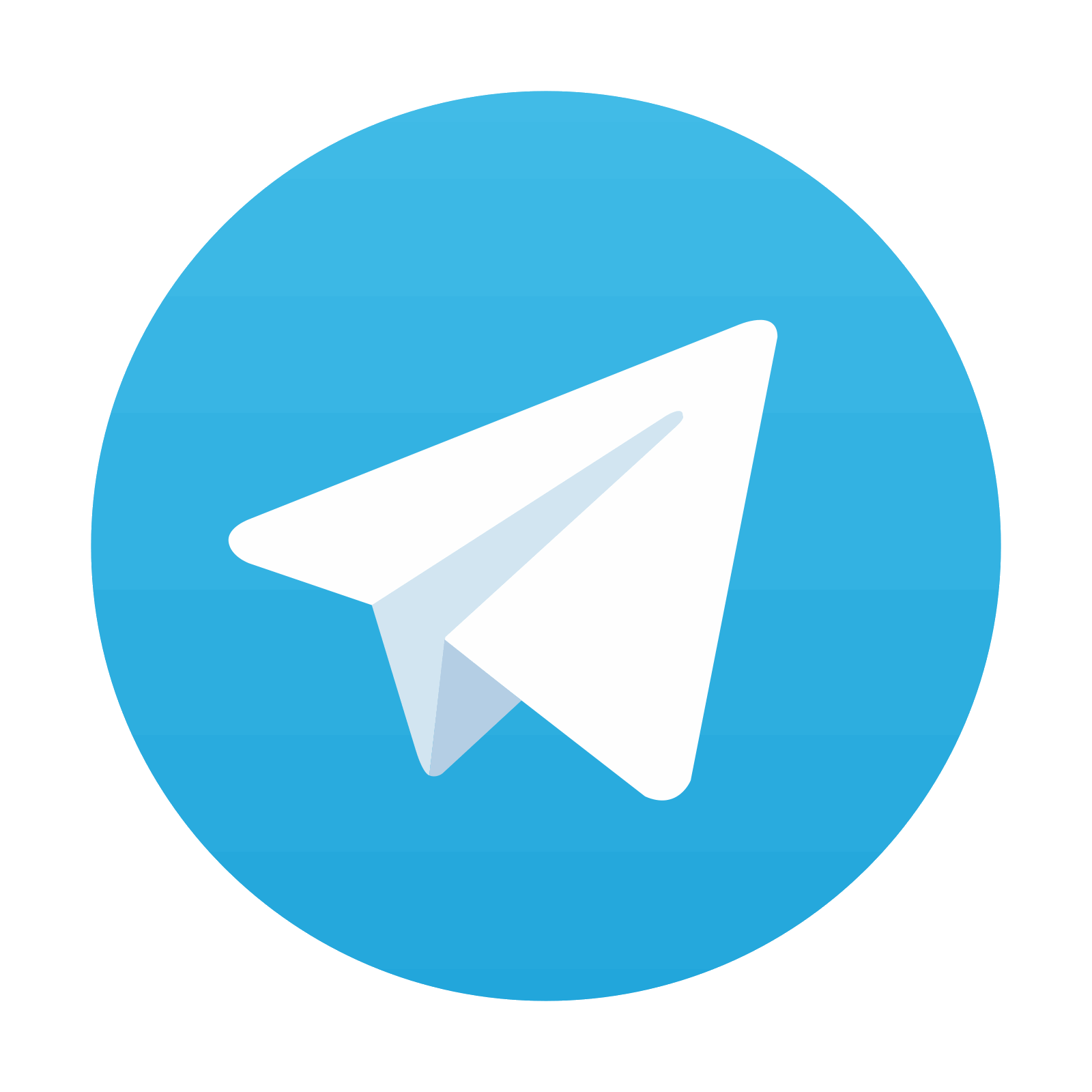
Stay updated, free articles. Join our Telegram channel
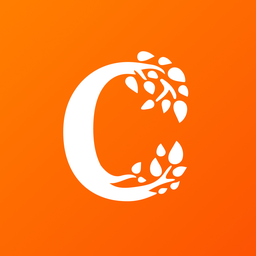
Full access? Get Clinical Tree
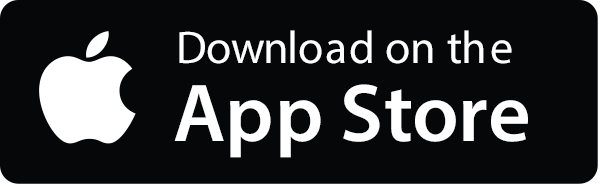
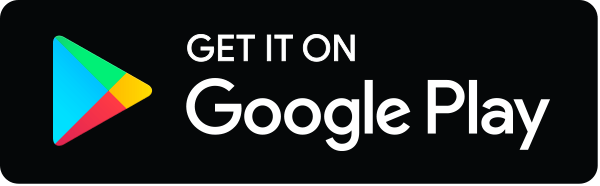