FIGURE 7.1 Computed tomographic (CT) scan of an 18-month-old with large left epidural hematoma. Note the swirling nature of the hematoma indicating active bleeding.
Subdural hematomas: An accumulation of blood between the dura and the brain parenchyma, usually the result of injury to the cortical bridging veins. They are more common in infancy and the incidence decreases with age (23). Acute subdural hematomas are usually the result of high velocity injuries and are associated with primary brain parenchyma injuries with tearing of bridging veins such as contusions or TAI (24). In infants without a clear history or mechanism for accidental trauma or with concurrent interhemispheric bleeding, further evaluation for inflicted trauma is required.
Subarachnoid hemorrhage: An accumulation of blood within the subarachnoid space. Subarachnoid hemorrhage occurs after injury to the small vessels traversing the subarachnoid space. It does not typically require emergent intervention, unless it causes cerebrospinal fluid (CSF) flow obstruction leading to hydrocephalus, which suggests there may be associated significant brain parenchymal injury.
Intraparenchymal hemorrhage: Hemorrhagic contusions are common after pediatric head injury (Figure 7.2), but large intracerebral hemorrhages are rare (25). Contusions result from the brain impacting the adjacent skull.
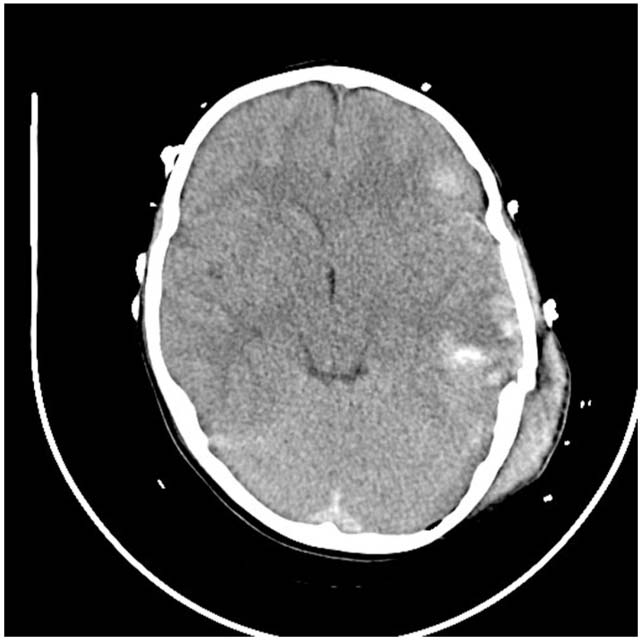
FIGURE 7.2 CT scan of 10-year-old struck by automobile with multiple parenchymal contusions.
■ EVALUATION
History
Details on the timing and mechanism of injury, resuscitative efforts, and therapeutic interventions implemented and attempted from witnesses at the scene as well as from emergency medical personnel are essential. The use of the “AMPLE” mnemonic (allergies, medications currently used, past illnesses, last meal, and events/environment related to the injury) may be helpful for practitioners to quickly acquire the necessary information to improve understanding of the patient’s current physiologic state (26). Presentation symptoms have little or no correlation with injury severity and the practitioner must rely on physical examination (27).
Physical Examination
Adequacy of the ABCs and the patient’s neurologic status must be rapidly assessed. Immediate evaluation for life-threatening signs and symptoms of intracranial hypertension or impending herniation is essential. These symptoms include altered level of consciousness, pupillary dysfunction, lateralizing extremity weakness, Cushing triad (hypertension, bradycardia, and irregular respirations), or herniation syndromes (Table 7.1).
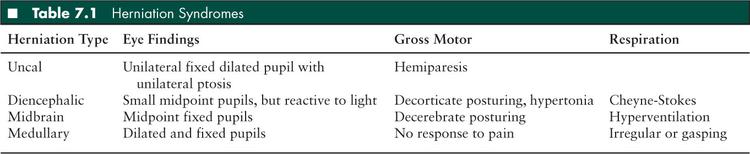
If the patient is intubated, capnography permits rapid assessment for esophageal intubation (28–30) as well as optimization of arterial carbon dioxide. The head and spine should be thoroughly examined for any external evidence of injury such as scalp lacerations and skull depressions that may indicate underlying skull fracture and severe intracranial injury. In the infant, a bulging fontanelle may be a sign of increased intracranial pressure (ICP), although a flat fontanelle does not preclude intracranial hypertension (6). Mastoid (Battle sign) and periorbital (raccoon eyes) bruising due to dissection of blood, hemotympanum, and clear rhinorrhea are all signs of possible basilar skull fracture. A basilar skull fracture would be a contraindication to a nasal tube.
It is important that a rapid but detailed and easily reproducible neurologic assessment be performed and documented (27). The Glasgow Coma Scale (GCS) is the most widely used method to quantify initial neurologic assessment in older children and adults (Table 7.2) (31). In younger pediatric patients, the Children’s Coma Scale is often utilized (Table 7.3) (32). It is critical that the GCS be recorded on the initial medical record and repeated regularly to detect changes in GCS indicative of progressive injury.
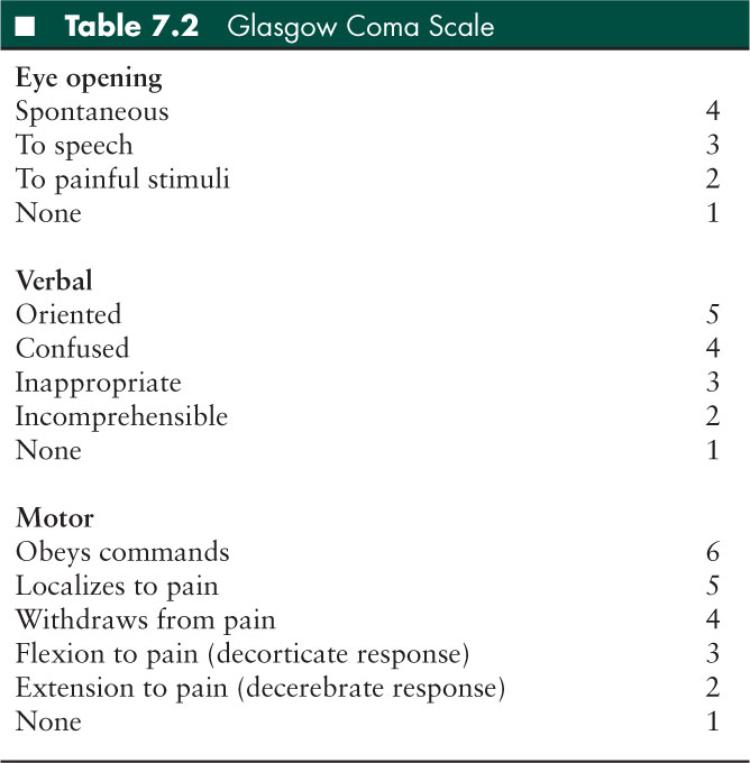
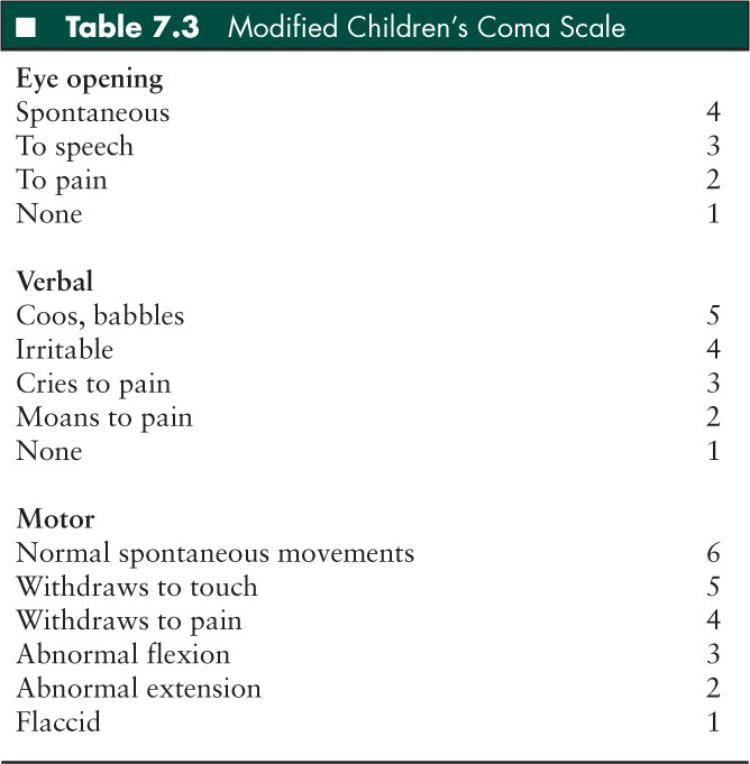
The pupillary exam is vital when assessing the neurologic status of the head-injured child. The size, shape, and reactivity to light provide critical insight into the balance of sympathetic and parasympathetic influences. Evaluation of eye movements and brainstem reflexes can help localize intracranial lesions. Dysfunction of all three cranial nerves in eye movement (oculomotor, trochlear, and abducens nerves) can be the result of injury to the ipsilateral cavernous sinus. Cough and gag reflexes detect glossopharygneal and vagus nerve function. Abnormalities in the respiratory pattern may also assist in localizing brain injury and herniation syndromes (Table 7.1). Deep tendon reflexes (DTRs) are typically exaggerated in head-injured patents due to the lack of cortical inhibition. However, decreased DTRs may suggest a spinal cord injury. The presence of Cushing triad (systemic hypertension, bradycardia, and irregular respirations) due to compression of the cardiorespiratory centers in the brainstem is a late and ominous sign for herniation.
Diagnostic Studies
The ABCs are first addressed with a chest x-ray to rule out any associated thoracic injuries and appropriate endotracheal tube placement. Likewise, the spine is immobilized presumptively until those injuries are ruled out. Computed tomography (CT) is the mainstay of the diagnostic evaluation of the severely head-injured pediatric patient. For intubated patients, continuous capnometry is vital for titrating treatment of intracranial hypertension (see below). A chemistry panel should be sent to assess electrolyte abnormalities and renal function, especially if hyperosmolar therapy may be instituted (33). Liver enzymes and pancreatic function should also be evaluated for possible blunt trauma and especially if nonaccidental trauma is suspected. A complete blood count and a coagulation profile should be evaluated in patients with intracranial hemorrhage. In one prospective observational study, 22% of children with severe head injury had laboratory evidence of disseminated intravascular coagulation (34). Repeated measurements may be needed since coagulopathy or thrombocytopenia may develop over time (35). In the adolescent population, where there are increased rates of high impact injuries from motor vehicle crashes, a toxicology screen should also be considered (36).
Imaging with plain films of the cervical spine should be performed to assess for associated cervical spine injury or instability that may require urgent immobilization and management. Chest radiographs may identify rib fractures, pneumothoracis, and widened mediastinum, and are needed for intubated patients to evaluate for right mainstem intubations. Pelvic radiographs should be considered if pelvic injuries are suspected. Other radiographs should be performed based on the results of the secondary survey. If nonaccidental injury is suspected then a full skeletal survey may be indicated. If there is no clear history or mechanism of accidental trauma, especially in infants and early toddlers, further investigation for other occult injuries, such as abdominal injuries, skeletal injuries, or retinal hemorrhages (which are commonly associated with shaken-baby or shaken-impact syndrome) should be sought (37). However, this workup should not take precedence over life-threatening issues such as hypoxemia, hypotension, and intracranial hypertension.
In pediatric patients with moderate and severe TBI, CT scans are the imaging modality of choice and can rapidly detect skull fractures, intracranial hematomas, intraparenchymal contusions, cerebral edema, and obliteration of the basal cisterns. CT scan has widespread availability and rapid imaging time which makes it very useful in the emergency and critical care settings. Several early CT scan findings have been associated with outcome including the status of the basal cisterns, midline shift, and presence of subarachnoid hemorrhage in the basal cisterns (38–40). The basal cisterns are evaluated at the level of the midbrain—compressed or absent cisterns increase the risk of intracranial hypertension and poor outcome (40,41). The degree of midline shift at the foramen of Monro is inversely related to prognosis (38, 40,41). The presence of traumatic subarachnoid hemorrhage in severe TBI increases mortality and its presence in the basal cisterns is a predictor of poor outcome (38, 40,41).
Magnetic resonance imaging (MRI) has demonstrated superiority to CT for detection of TAI and its correlation with long-term outcome. However, MRI is of limited use during the initial stabilization and management of pediatric TBI patients due to limited availability, long time required for image acquisition, and limited physiologic monitoring availability in the MRI suite (42–44). In summary, pediatric patients with moderate and severe TBI should have CT imaging as soon as possible, but not before physiologic stabilization has occurred (ABCs) and appropriate monitoring has been placed (such as end-tidal CO2).
■ MANAGEMENT
Initial Stabilization
Hypoxemia and hypotension should be avoided and managed to prevent or minimize secondary hypoxic-ischemic brain injury, which may promote cerebral swelling and elevated ICP. Thus, the first step in treating the head-injured pediatric patient is always to promote adequate oxygenation and ventilation, and to prevent or treat shock.
Criteria for tracheal intubation include: hypoxemia not resolved with supplemental oxygen, apnea, hypercarbia (Paco2 > 45 mmHg), GCS ≤ 8, a decrease in GCS > 3 independent of the initial GCS, anisocoria > 1 mm, cervical spine injury compromising ventilation, loss of pharyngeal reflex, and any clinical evidence of a herniation syndrome or Cushing triad (6).
Intubation should be performed utilizing a cerebroprotective, rapid-sequence induction and all patients should be assumed to have a full stomach and cervical spine injury. Supplemental oxygen should be delivered by face mask to allow nitrogen washout from the patient’s functional residual capacity to allow sufficient oxygenation prior to intubation of the trachea. Bag-valve-mask ventilation should not be done, unless the patient has signs and symptoms of impending herniation, apnea, or hypoxemia (45). As a general rule, the proper tracheal tube size for children older than 6 months of age (as described by the inner diameter measured in millimeters) = (16 + age [in years]) divided by 4, but this is only a starting estimate. Tracheal tubes that are one size smaller and larger should always be available. Orotracheal intubation by direct laryngoscopy is the preferred method. Nasotracheal intubation should be avoided since it may require excessive movement of the cervical spine and could result in direct intracranial damage in a patient with a basilar skull fracture. The endotracheal tube should be secured with tape, but this adhesive tape should not pass around the neck as venous return from the brain can be compromised.
Because endotracheal intubation is a noxious stimulus and can increase ICP, appropriate medications should be used during rapid-sequence induction. A combination of medications to sedate and paralyze the patient are required. Medications and doses must be chosen to ensure intubation occurs quickly and safely and episodes hypoxemia and hypotension are avoided. The hemodynamic and neurologic status of the patient dictates drug choice. For the patient in cardiopulmonary arrest, no medications are needed for tracheal intubation. All the other patients usually receive lidocaine (1–1.5 mg/kg) intravenously (IV) 3 minutes before intubation to help blunt the rise in ICP that occurs during direct laryngoscopy (46). For the hemodynamically unstable patient, the combination of lidocaine, etomidate (0.2–0.6 mg/kg), and neuromuscular blockade with rocuronium (1 mg/kg) or vecuronium (0.3 mg/kg) IV is a popular choice. An alternative is the combination of lidocaine, fentanyl (2–4 micrograms/kg), and rocuronium or vecuronium. In the hemodynamically stable patient, either of the above combinations in addition to the fast-acting benzodiazepine midazolam (0.1–0.2 mg/kg) can utilized. Another alternative in the hemodynamically stable patient is the combination of thiopental (3–5 mg/kg), lidocaine, and rocuronium or vecuronium. Thiopental and etomidate are ultrafast acting and quickly reduce cerebral metabolism, which ameliorates the increased ICP associated with direct laryngoscopy. In addition, the short-acting narcotic fentanyl, when used with lidocaine, can decrease the catecholamine release associated with direct laryngoscopy (45).
Unless the patient has signs or symptoms of herniation and a more definitive therapy such as neurosurgical intervention is being instituted, prophylactic hyperventilation (Paco2 < 35 mmHg) should be avoided. Hyperventilation causes cerebral vasoconstriction, which decreases CBF and subsequent cerebral blood volume. Thus, while ICP may be reduced temporarily, ischemia can occur (47), and this could lead to further secondary injury and ICP elevation. Furthermore, respiratory alkalosis caused by hyperventilation shifts the hemoglobin-oxygen curve to the left, leading to less oxygen release in the brain.
Assessment of the patient’s circulatory status (central and peripheral pulse quality, capillary refill, heart rate, blood pressure) is critical as hypotension after pediatric TBI is associated with increase in morbidity and mortality (1,48,49). The most common cause for compensated or “early” shock (tachycardia with normal blood pressure) and uncompensated or “late” shock (low blood pressure) in the trauma patient is hypovolemic (ie, hemorrhagic) shock. Rapid IV fluid resuscitation is needed for hypovolemic shock. Isotonic solutions, such as 0.9% NaCl solution and/or packed red blood cells can be administered. Hypotonic fluids can worsen cerebral edema and should be avoided. Although its efficacy has not been studied in a clinical trial, resuscitation with hypertonic saline (3% saline) is generally indicated in the severely brain injured child with initial signs and symptoms of both hypovolemic shock and intracranial hypertension, and is further discussed in the “Intracranial Hypertension Management: First-Tier Therapies” section below. If hypotension is present and cervical-thoracic spine injuries are present or suspected then special consideration must be given to spinal (neurogenic) shock. These patients may also be bradycardic. Both spinal shock and bradycardia can be treated with isotonic fluid and/or blood resuscitation to ensure adequate circulation and prevent further ischemia. In spinal shock, α-adrenergic agonists, such as IV phenylephrine, are also needed to treat the vasodilatation that results from injury to the sympathetic outflow tract.
Prophylactic “brain-specific” interventions (such as hyperventilation and hyperosmolar therapy with mannitol or 3% saline) in the absence of signs and symptoms of herniation or other neurologic deterioration, are not recommended. However, in the presence of signs and symptoms of herniation, such as Cushing triad (irregular respirations, bradycardia, systemic hypertension), pupillary dysfunction, lateralizing extremity weakness, or extensor posturing, emergent treatment is needed and is discussed further in the “Herniation” section below.
Herniation
While the ABCs are being addressed, signs and symptoms of impending herniation such as Cushing triad or one of the herniation syndromes must also be immediately treated. Early consultation with a neurosurgeon is important. In one study, mass lesions occurred in 30% of children with severe TBI (50). Hyperventilation with 100% oxygen can be lifesaving in the setting of impending herniation, such as a child who has a rapidly expanding epidural hematoma with pupillary dilatation, bradycardia, systemic hypertension, and extensor posturing. Elevating the head to 30° increases venous drainage and lowers ICP (51). Furthermore, the head should be midline to prevent obstruction of venous return from the brain. If these maneuvers do not relieve the signs and symptoms of herniation, hyperosmolar therapy (mannitol or 3% saline) should be instituted (see the “Intracranial Hypertension Management: First-Tier Therapies” section below). In addition, intubating doses of short acting medications, such as thiopental or etomidate, as described previously, can be administered emergently in this setting (6). During this time, the patient usually undergoes CT imaging and/or is taken directly to the neurosurgical operating room for definitive therapy. Surgical evacuation of hemorrhagic lesions or craniotomy to reduce ICP may terminate herniation and be lifesaving.
Besides expanding mass lesions, diffuse cerebral swelling may also lead to herniation. Diffuse swelling is more common in pediatric than adult TBI (52). As a result, secondary causes of brain injury, such as hypoxemia, hypercarbia, hypotension, excessive fluid administration (especially hypotonic fluids), or seizures that can precipitate herniation must be prevented or immediately treated. Craniotomy may be useful for severe cerebral edema, especially if it is focal or hemispheric.
ICP, Autoregulation, and Cerebral Perfusion
Once the initial resuscitation with attention to the ABCs is completed and issues of herniation or expanding hemorrhages have been medically and surgically addressed, further management is aimed at preventing or treating causes of secondary brain injury. These include hypoxemia, hypotension, intracranial hypertension, hypercarbia, hyperglycemia or hypoglycemia, electrolyte abnormalities, enlarging hematomas, coagulopathy, seizures, and hyperthermia.
One of the most important consequences of secondary brain injury is the development of intracranial hypertension. First described in the Monroe-Kellie doctrine, the intracranial vault is a fixed volume of brain, CSF, and blood (6). An enlarging space occupying lesion, such as an expanding epidural hematoma or worsening cerebral edema, will not initially cause intracranial hypertension since initial compensatory mechanisms including displacement of CSF to the spinal canal and venous blood to the jugular veins is sufficient to prevent elevated ICP. However, once these compensatory mechanisms are exhausted, even a small increase in the size of the hematoma or cerebral edema will lead to increased ICP. As ICP approaches mean arterial pressure (MAP), cerebral perfusion is compromised. This leads to ischemia which worsens cerebral edema, further elevating ICP and reducing cerebral perfusion, and this cycle may ultimately lead to herniation.
Under normal conditions, cerebral autoregulation provides constant CBF over a wide range of CPPs and is “coupled” to the metabolic demands of the brain. CPP is the defined as the difference between MAP and ICP. Thus, CPP = MAP – ICP (6). After TBI, cerebral autoregulation can become “uncoupled” from the metabolic demands of the brain. Without autoregulation, reduction in CPP due to either elevated ICP or reduced MAP may result in CBF fluctuations, potentially leading to cerebral ischemia. In contrast, increased CPP due to a decrease in ICP or increased MAP may increase CBF and result in hyperemia. A study utilizing xenon CBF-CT studies in children after TBI demonstrated that marked reductions in CBF within the first 24 hours after injury was associated with poor outcome, while high CBF 24 hours after the injury was associated with improved outcome (8). However, because this type of study cannot measure minute-to-minute assessment of CBF fluctuations, and due to the potential risk of transporting and performing prolonged studies in critically-ill patients, most institutions continuously measure ICP and MAP to calculate CPP, which is used as an estimate of CBF.
In general, after initial stabilization and resuscitation, an ICP monitor is placed by the neurosurgeon in children with an initial GCS ≤ 8 for treatment of potential intracranial hypertension. Since clinical signs and symptoms of herniation are very late signs of intracranial hypertension, the use of ICP monitors allows early detection of intracranial hypertension, often well before signs and symptoms of herniation are observed (53). An additional benefit is that if the ventricles are not compressed due to severe cerebral swelling, ICP monitoring by ventricular catheter permits therapeutic CSF drainage if needed. However, ICP monitors can rarely cause hemorrhage and infection. Coagulopathy needs to be corrected before ICP monitor placement and some centers administer prophylactic antibiotics. Placement of an intracranial monitor should be performed with adequate anesthesia.
Treatment for intracranial hypertension should begin at an ICP ≥ 20 mmHg, as most pediatric TBI studies show poor outcome with ICP > 20 mmHg, and aggressive treatment of intracranial hypertension is associated with improved outcomes in some studies (54–57). However, further studies are needed to determine an age-appropriate treatment for intracranial hypertension in infants and young children. Younger children have a lower MAP, and thus may require a lower ICP treatment threshold if CPP is to be maintained. The optimal CPP for pediatric TBI is unknown and there is no evidence that targeting a specific CPP improves outcome. However, there are pediatric TBI studies that show that a CPP < 40 mmHg is associated with poor outcome (58,59). As a result, the pediatric guidelines recommend the option of maintaining an “age-related continuum” of CPP from 40 to 65 mmHg in infants to adolescents, respectively (1). Further studies are needed to determine the age-appropriate CPP.
Lowering ICP or raising MAP will increase CPP. Most treatments are aimed at lowering ICP, maintaining normal MAP, and maintaining euvolemia. If the treatments fail to lower ICP, then vasopressors are commonly added to augment MAP and thus increase the CPP. However, this mechanism only works if cerebral autoregulation is at least partially intact. Otherwise, as the MAP is increased, the ICP will also increase, and there is no net augmentation in CPP. If the child is hypotensive, isotonic fluid boluses and/or vasopressors can be administered to augment the MAP in the hopes of improving CPP.
Intracranial Hypertension Management: First-Tier Therapies
A general approach to first-tier treatments for established intracranial hypertension in pediatric TBI was provided in the “Guidelines for the management of severe TBI in infants, children, and adolescents” (1). As discussed below, first-tier therapies include head position; sedation, analgesia, and neuromuscular blockade; ventricular CSF drainage; hyperosmolar therapy; and mild hyperventilation.
Head Position
Elevating the head to 30° has been shown to reduce ICP without decreasing CPP in adults after severe TBI (51). While pediatric studies are lacking, the same degree of head elevation with midline position may promote venous drainage. There are some patients who may have a more optimal CPP at angles other than 30º. This angle will change day to day, and may be greater or less than 30º. Optimally, the head position will be individualized and titrated to CPP or CBF monitors (60). Head elevation may also reduce ventilator associated pneumonias. In some centers, practitioners avoid placing a central venous catheter in the internal jugular vein to maximize venous drainage from the brain.
Sedation, Analgesia, and Neuromuscular Blockade
If there is continued ICP elevation, sedation, analgesia, and neuromuscular blockade can be administered. Anxiety, stress, and pain can increase cerebral metabolic demands, which can pathologically increase cerebral blood volume and increase ICP. Narcotics, benzodiazepines, or barbiturates are commonly used. There are few studies of the use of sedatives in pediatric patients with severe TBI. However, the goal should be to use the lowest possible doses to lower ICP without causing side effects such as hypotension. In addition, potentially noxious stimulus such as endotracheal tube suctioning should be pretreated with sedation and/or analgesics, and lidocaine (1 mg/kg IV) may be utilized to blunt rises in ICP.
Ketamine has been associated with increasing cerebral blood volume and increased ICP, and thus has been considered contraindicated in patients with increased ICP (61). More recently, ketamine administration in mechanically ventilated pediatric TBI patients has been reported to decrease ICP (62). Further study of ketamine is needed.
A number of non-TBI and one TBI case report have reported metabolic acidosis and death in pediatric patients on prolonged (24 hours) continuous infusion of propofol (63–66). Based on recommendations of the Food and Drug Administration, the pediatric guidelines state: “continuous infusion of propofol is not recommended in the treatment of pediatric traumatic brain injury” (1).
Neuromuscular blocking agents are thought to reduce ICP by reducing airway and intrathoracic pressure with improved cerebral venous outflow and by preventing shivering, coughing, posturing, or ventilator-patient asynchrony (67). Risks of neuromuscular blockade include hypoxemia and hypercarbia due to inadvertent extubation, masking of seizures, nosocomial pneumonia (shown to occur in adults with severe TBI), immobilization stress due to inadequate sedation and analgesia, increased ICU stay duration, and myopathy of critical illness (67). The loss of clinical exam should be less concerning if ICP monitoring is used, as increases in ICP usually occur before changes in clinical exam.
Ventricular Cerebrospinal Drainage
Removal of CSF may decrease ICP in a patient with intracranial hypertension. The placement of a ventricular ICP monitor provides diagnostic information as well as a therapeutic intervention (CSF drainage). Small ventricles due to diffuse cerebral edema or intracranial bleeding may make catheter placement difficult.
Hyperosmolar Therapy
Two agents are generally considered for hyperosmolar therapy: mannitol and 3% normal saline. While mannitol has been traditionally administered, 3% saline is also gaining favor. However, there is no literature to support the superiority of one over the other in severe pediatric TBI. Mannitol reduces ICP by two mechanisms. First, mannitol rapidly reduces blood viscosity which promotes reflex vasoconstriction of the arterioles by autoregulation, thus decreasing cerebral blood volume and ICP. In addition to decreasing intracranial blood volume, it may enhance flow dependent vasodilation, improving CBF overall. This mechanism is rapid but transient, lasting about 75 minutes, and it requires an intact autoregulation (68,69). Second, mannitol has an osmotic effect. By increasing serum osmolality, mannitol causes a shift of water from the brain cells into the intravascular space, and thus decreases cellular or cytotoxic edema. While this effect is slower in onset (15–30 minutes), the osmotic effect lasts up to 6 hours. Importantly, if the blood-brain barrier is not intact, which is often the case in children with severe TBI, over time mannitol may accumulate in injured brain regions and cause a shift in fluid from the intravascular space to the brain parenchyma and thus worsen ICP. This problem is reported when mannitol is present in the circulation for extended periods, supporting the use of intermittent boluses (70,71). Furthermore, mannitol is a potent osmotic diuretic and may precipitate hypotension and renal failure if the patient becomes hypovolemic and the serum osmolality is > 320 mOsm/L (72–74). Mannitol is administered in bolus doses of 0.25 g/kg to 1 g/kg IV (72).
More recently, hypertonic saline has been used for hyperosmolar therapy in pediatric TBI. The main mechanism of action is the osmotic effect similar to mannitol, but its main theoretical advantage over mannitol is that hypertonic saline can be administered in a hemodynamically unstable patient with impending herniation, as hypertonic saline is thought to preserve intravascular volume status (75–78). Mannitol is an osmotic diuretic and may worsen intravascular depletion and hypotension. Hypertonic saline exhibits several other theoretical benefits such as restoration of normal cellular resting membrane potential and cell volume, inhibition of inflammation, stimulation of atrial natriuretic peptide release, and enhancement of cardiac output (78–81). Hypertonic saline, as 3% saline, can be administered as a bolus IV dose. While not well studied, 1–6 mL/kg IV is an often used dose. Three percent saline continuous infusions of 0.1 to 1 mL/kg/hour titrated to maintain ICP < 20 mmHg have been used (82,83). Caution should be exercised if the serum osmolality approaches 320 mOsm/L as there have been reports of renal insufficiency (33). Another potential concern with the use of hypertonic saline is myelinosis, which may be central pontine (demyelination of the pons) or extrapontine (demyelination of the thalamus, basal ganglia, and cerebellum). Myelinosis occurs with hypernatremia and/or its correction (84), although this has not been clinically reported in pediatric TBI management. Rebound intracranial hypertension has been described with the use of hypertonic saline bolus administration or after stopping the continuous infusion (82,85). Further studies evaluating optimal dosing and long-term outcome are needed to compare mannitol administration with 3% saline.
Hyperventilation
Hyperventilation may rapidly lower ICP. However, without signs of herniation, mild or prophylactic hyperventilation (Paco2 < 35 mmHg) in children should be avoided. Mild hyperventilation (Paco2 30–35 mmHg) may be considered as a first-tier option for longer periods of intracranial hypertension refractory to all the above measures (sedation, analgesia, neuromuscular blockade, CSF drainage, and hyperosmolar therapy) (1). This rationale is based on studies that CBF may be decreased early following pediatric TBI and may be associated with poor outcome, and that prophylactic hyperventilation may cause further ischemia and hypoperfusion (47). Aggressive hyperventilation (Paco2 < 30 mmHg) may be considered as a second tier option in the setting of refractory intracranial hypertension. CBF, jugular venous oxygen saturation, or brain tissue oxygen monitoring to help identify cerebral ischemia is suggested (86,87).
Intracranial Hypertension Management: Second-Tier Therapies
Refractory intracranial hypertension occurs in as many as 42% of cases of severe pediatric TBI and is associated with mortality rates between 29% and 100% (88–91). Repeat CT scan should be performed to rule out a surgically correctable etiology for persistent, refractory intracranial hypertension. If there is no surgical lesion, then the guidelines recommend second-tier therapies, which includes aggressive hyperventilation, barbiturates, hypothermia, decompressive craniectomy, and lumbar CSF drainage (1). There is less data for these therapies compared to first-tier therapies.
Barbiturates
Barbiturates reduce ICP by decreasing the cerebral metabolic rate (92–94). An electroencephalogram (EEG) should be used to assess the cerebral metabolic response to barbiturate treatment. Pentobarbital or thiopental is often administered to achieve burst suppression on the EEG, although the optimal degree of burst suppression is unknown. Side effects include decreased cardiac output, decreased systemic vascular resistance, and hypotension (89). As a result, if high-dose barbiturate therapy is used to treat refractory intracranial hypertension, then appropriate hemodynamic monitoring and cardiovascular support is required.
Hypothermia
In animal studies of experimental TBI, hyperthermia has been shown to exacerbate neuronal cell death. Further, therapeutic hypothermia was found to be neuroprotective by ameliorating mechanisms of secondary brain injury, such as decreasing cerebral metabolism, inflammation, lipid peroxidation, and excitotoxicity (95). While most agree that hyperthermia should be avoided in children with severe TBI, the role of hypothermia is unclear. A recent randomized controlled clinical trial did not show any benefit to 24 hours of hypothermia and there was a trend toward poorer outcome in the hypothermia group (96). Until further clinical studies are done, moderate hypothermia is reserved for patients with persistent intracranial hypertension refractory to other medical interventions, if no medical contraindications exist (1). Potential complications associated with hypothermia include hypovolemia (due to cold-induced dieresis), electrolyte disturbances such as hypokalemia with consequent arrhythmias, coagulopathy, and increased susceptibility to infection.
Decompressive Craniectomy
The main goal of decompressive craniectomy is to reduce ICP and thus maintain CPP, thereby preventing herniation due to refractory cerebral swelling. This surgical option is considered a second-tier therapy for pediatric TBI patients with refractory intracranial hypertension and may be particularly appropriate in patients who have a potentially recoverable brain injury. Other indications for decompressive craniectomy include secondary clinical deterioration or evolving cerebral herniation syndrome within 48 hours of injury (1). Decompressive craniectomy should be considered in the treatment of severe TBI and refractory intracranial hypertension in infants and young children with nonaccidental head trauma, as these patients had improved survival and neurologic outcomes compared to those undergoing medical management alone (97). However, there are concerns that this procedure may exacerbate hemorrhage and cerebral edema formation, so further studies addressing the safety, efficacy, and impact on long-term outcome of this surgical procedure are needed.
Lumbar CSF Drainage
Although not commonly used, lumbar CSF drainage has been shown to be successful in treating refractory intracranial hypertension following pediatric TBI (98). However, to avoid the risk of herniation, the child must already have a functional ventriculostomy drain and open basal cisterns, and no mass effect or shift on concurrent CT. Care is required to avoid herniation as pressure below the brain is lowered by lumbar CSF drainage.
Seizures
Seizures should be aggressively treated as seizures can elevate ICP and induce metabolic distress (99), potentially leading to worsened secondary brain injury. Note that many seizures may not have any clinical correlate and may be nonconvulsive, also referred to as subclinical or electrographic only seizures. Children with TBI may have nonconvulsive seizures, even when not paralyzed, and these can only be detected using continuous EEG monitoring (100). Studies are needed to determine whether detection and management of nonconvulsive seizures improves outcome.
While prophylactic anticonvulsants may be considered a treatment option to prevent early posttraumatic seizures (occurring within 7 days following injury) in infants and young children, prophylactic anticonvulsants are not recommended for preventing late posttraumatic seizures (occurring after 7 days), as they have not been shown to improve outcome (101,102). If clinical seizures are detected, most would treat with standard anticonvulsants. If nonconvulsive seizures are detected, most neurologists would treat using standard anticonvulsants (103), although further study is needed.
■ CONCLUSIONS
Resuscitation of the child with severe TBI should focus on prompt stabilization and avoidance of hypoxemia and hypotension. Early evaluation to determine the need for surgical intervention is critical. Care in the ICU setting should focus on the prevention or amelioration of secondary brain injury including optimization of CPP and reduction of intracranial hypertension. Further research is needed on the optimization of pediatric neurointensive care for the child with severe TBI, as well as the development of novel therapeutics aimed at the cellular and subcellular level to promote neuron recovery.
■ REFERENCES
1. et al. Guidelines for the acute medical management of severe traumatic brain injury in infants, children, and adolescents. Pediatr Crit Care Med. 2003;4:S1–S75.
2. , . Brain Injury in the United States: Emergency Department Visits, Hospitalizations, and Deaths. Centers for Disease Control and Prevention, National Center for Injury Prevention and Control. Atlanta, GA; 2004:1–32.
3. et al. Incidence of pediatric traumatic brain injury and associated hospital resource utilization in the United States. Pediatrics. 2006;118(2):483–492.
4. et al. A population-based study of inflicted traumatic brain injury in young children. JAMA. 2003;290:621–626.
5. . Pediatric traumatic brain injury. Crit Care Nursing. 1997;20:36–51.
6. et al. Severe traumatic brain injury in infants and children. In: , , eds. Pediatric Critical Care. Philadelpia: Mosby; 2006:1595–1617. Textbook.
7. et al. Overview of basic mechanisms underlying neuropathological consequences of head trauma. In: , , eds. Head Trauma—Basic, Preclinical, and Clinical Directions. Hoboken, NJ, Wiley-Liss; 2001:3–36. Review.
8. et al. Cerebrovascular response in infants and young children following severe traumatic brain injury: a preliminary report. Pediatr Neurosurg. 1997;26:200–207.
9. . Cerebral hemodynamics after traumatic brain injury of immature brain. Exp Toxicol Pathol. 1999;51:137–142.
10. . Superoxide generation links protein kinase C activation to impaired ATP sensitive K-channel function after brain injury. Stroke. 1999;30:153–159.
11. . Brain injury impairs prostaglandin cerebrovasodilation. J Neurotrauma. 1998;15:721–729.
12. . Role of endothelin-1 in age-dependent cerebrovascular hypotensive responses after brain injury. Am J Physiol. 1999;277:111884–111894.
13. . Ionic dependence of glutamate neurotoxicity. J Neurosci. 1987;7:369–379.
14. et al. Biochemical, cellular, and molecular mechanisms in the evolution of secondary damage after severe traumatic brain injury in infants and children: Lessons learned from the bedside. Pediatr Crit Care Med. 2000;1(1):4–19.
15. et al. Contribution of vasogenic and cellular edema to traumatic brain swelling measured by diffusion-weighted imaging. J Neurosurg. 1997;87(6):900–907.
16. , . The pathobiology of traumatically induced axonal injury in animals and humans: a review of current thoughts. In: , , , eds. Traumatic Brain Injury: Bioscience and Mechanics. New York, NY: Mary Ann Liebert; 1996:51–60. Review.
17. et al. Diffuse axonal injury due to nonmissile head injury in humans: an analysis of 45 cases. Ann Neurol. 1982;12(6):557–563.
18. et al. [Diffuse axonal lesions in childhood]. Pediatr Med Chir. 1998;20(6):393–397.
19. et al. Axonal injury and the neuropathology of shaken baby syndrome. Acta Neuropathol (Berl). 1998;95(6):625–631.
20. et al. Metabolic and clinical markers of prognosis in the era of CT imaging in children with acute epidural hematomas. Pediatr Neurosurg. 2000;33(2):70–75.
21. et al. Extradural hematomas in children. J Neruosurg Sci. 1998;42(2):95–99.
22. et al. Prognosis of extradural hematomas in children. Pediatr Neurosurg. 1995;23(2):57–63.
23. et al. Head injuries in children under 36 months of age. Demography and outcome. Childs Nerv Syst. 1988;4(1):34–40.
24. , , . Acute subdural hematomas: review of 144 cases. J Neurosurg. 1975;42:37.
25. et al. Traumatic isolated intracerebral hemorrhage in children. Childs Nerv Syst. 1989;5(5):303–306.
26. ATLS: Advanced Trauma Life Support for Doctors. 7th ed. Chicago, IL: American College of Surgeons; 2004. Textbook.
27. et al. Management and classification of children with head injury. Childs Nerv Syst. 20005;21(6):430–436.
28. et al. Use of capnography in the delivery room for assessment of endotracheal tube placement. J Perinat. 2001;21(5):284–287.
29. et al. The use of capnography for recognition of esophageal intubation in the neonatal intensive care unit. Pediatr Pulmonol. 1995;19(5):262–268.
30. et al. The effectiveness of out-of-hospital use of continuous end-tidal carbon dioxdie monitoring on the rate of unrecognized misplaced intubation within a regional emergency medical services system. Ann Emerg Med. 2005;45:497–503.
31. , . Assessment of coma and impaired consciousness. A practical scale. Lancet. 1974;13:81–84.
32. et al. Assessing the conscious level in infants and young children: a pediatric version of the Glasgow Coma Scale. Childs Nerv Syst. 1988;4(1):30–33.
33. , , . Caution should be exercised when maintaining a serum sodium level >160 meq/L. Crit Care Med. 2004;32(6):1438–1439.
34. et al. The influence of hemocoagulative disorders on the outcome of children with head injury. Pediatr Neurosurg. 2001;34:131–137.
35. et al. Subsequent development of thrombocytopenia and coagulopathy in moderate and severe head injury: support for serial laboratory examination. J Trauma. 2005;58:725–730.
36. , . Epidemiology and outcomes of pediatric traumatic brain injury. Dev Neurosci. 2006;28:256–263.
37. et al. Nonaccidental head injury in infants—the “shaken-baby syndrome”. N Engl J Med. 1998;338:1822–1829.
38. et al. Predictive value of initial computerized tomography scan, intracranial pressure, and state of autoregulation in patients with traumatic brain injury. J Neurosurg. 2006;104(5):731–737.
39. et al. Prognostic factors in children with severe diffuse brain injuries: a study of 74 patients. Pediatr Neurosurg. 2001;34(2):98–103.
40. et al. Appendix II: evaluation of relevant computed tomographic scan findings. Neurosurgery. 2006;58(3):S62.
41. et al. Prognostic value of computerized tomography scan characteristics in traumatic brain injury: results from the IMPACT study. J Neurotrauma. 2007;24(2):303–314.
42. et al. Susceptibility-weighted imaging and proton magnetic resonance spectroscopy in assessment of outcome after pediatric traumatic brain injury. Arch Phys Med Rehabil. 2006;87:850–858.
43. , , . Use of advanced neuroimaging techniques in the evaluation of pediatric traumatic brain injury. Dev Neurosci. 2006;28:309–326.
44. et al. Diffusion tensor imaging in the corpus callosum in childre n after moderate to severe traumatic brain injury. J Neurotrauma. 2006;23:1412–1426.
45. , . Traumatic head or spinal injury. In: , ed. Textbook of Pediatric Transport Medicine. Philadelphia, PA: Mosby; 1995.
46. , . Prophylactic lidocaine use preintubation: a review. J Emerg Med. 1994;12(4):499–506.
47. et al. Effect of hyperventilation on regional cerebral blood flow in head-injured children. Crit Care Med. 1997;25(8):1402–1409.
48. et al. Influence of definition and location of hypotension on outcome following severe pediatric traumatic brain injury. Crit Care Med. 2005;33(11):2645–2650.
49. et al. Epidemiology and early predictive factors of mortality and outcome in children with traumatic severe brain injury: experience of a French pediatric trauma center. Pediatr Crit Care Med. 2006;7(5):461–467.
50. Anderson VA et al. Identifying factors contributing to child and family outcome 30 months after traumatic brain injury in children. J Neurol Neurosurg Psychiatry. 2005;76(3):401–408.
51. et al. Effect of head elevation on intracranial pressure, cerebral perfusion pressure, and cerebral blood flow in head-injured patients. J Neurosurg. 1992;76(2):207–211.
52. et al. Diffuse brain swelling in severely head-injured children. A report from the NIH Traumatic Coma Data Bank. J Neurosurg. 1992;76(3):450–454.
53. . Continuous recording and control of ventricular fluid pressure in neurosurgical practice. Acta Psychiatr Scand Suppl. 1960;36(149):1–193.
54. et al. Treatment and outcome of the severely head injured child. Intensive Care Med. 1983;9(1):13–16.
55. et al. Outcome in children with severe head injuries. Childs Nerv Syst. 1985;1(2):109–114.
56. et al. Cerebral blood flow and metabolism in children with severe head injury. Part 1: relation to age, Glasgow Coma Score, outcome, intracranial pressure, and time after injury. J Neurol Neurosurg Psychiatry. Feb 1995;58(2):145–152.
57. , . Clinical applications of the pressure-volume index in treatment of pediatric head injuries. J Neurosurg. 1982;56(6):819–825.
58. et al. Management and outcome of severe head injuries in the Trent region 1985-90. Arch Dis Child. 1992;67:1430–1435.
59. et al. Relationship of cerebral perfusion pressure and survival in pediatric brain-injured patients. J Trauma. 2000;49:654–658.
60. et al. Influence of body position on jugular venous oxygen saturation, intracranial pressure and cerebral perfusion pressure. Acta Neurochir Suppl (Wien). 1993;59:107–112.
61. . Ketamine. Mt Sinai J Med. 1983;50(4):300–304.
62. et al. Effectiveness of ketamine in decreasing intracranial pressure in children with intracranial hypertension. J Neurosurg Pediatr. 2009;4(1):40–46.
63. . Propofol infusion syndrome in children. Paediatr Anaesth. 1998;8(6):491–499.
64. , , . Lactic acidemia and bradyarrhythmia in a child sedated with propofol. Crit Care Med. 1998;26(12):2087–2092.
65. et al. Metabolic acidosis and fatal myocardial failure after propofol infusion in children: five case reports. BMJ. 1992;305(6854):613–616.
66. et al. Massive ketonuria during sedation with propofol in a 12 year old girl with severe head trauma. Acta Anaesthesiol Belg. 1994;45(1):19–22.
67. et al. Early, routine paralysis for intracranial pressure control in severe head injury: is it necessary? Crit Care Med. 1994;22(9):1471–1476.
68. , . Effect of mannitol on ICP and CBF and correlation with pressure autoregulation in severely head-injured patients. J Neurosurg. 1984;61(4):700–706.
69. et al. Mannitol causes compensatory cerebral vasoconstriction and vasodilation in response to blood viscosity changes. J Neurosurg. 1983;59(5):822–828.
70. . Methodology for the control of intracranial pressure with hypertonic mannitol. Acta Neurochir (Wien). 1980;51(3-4):161–172.
71. , . Aggravation of vasogenic cerebral edema by multiple-dose mannitol. J Neurosurg. 1992;77(4):584–589.
72. , , , , , , , , , , . Management and prognosis of severe traumatic brain injury. part 1: guidelines for the management of severe traumatic brain injury. Use of mannitol. J Neurotrauma. 2000;17:521–525.
73. , . The alleviation of increased intracranial pressure by the chronic administration of osmotic agents. In: , , eds. Intracranial Pressure. Berlin, Germany: Springer; 1972:309–315.
74. , . The hypertonic state. N Engl J Med. 1977;297(26):1444–1454.
75. et al. Regional cerebral blood flow following resuscitation from hemorrhagic shock with hypertonic saline. Influence of a subdural mass. Anesthesiology. 1991;75(2):319–327.
76. et al. Hypertonic saline resuscitation of patients with head injury: a prospective, randomized clinical trial. J Trauma. 1998;44(1):50–58.
77. , , . A comparison of hypertonic to isotonic fluid in the resuscitation of brain injury and hemorrhagic shock. J Surg Res. 1991;50(3):284–292.
78. , . Use of hypertonic saline solutions in treatment of cerebral edema and intracranial hypertension. Crit Care Med. 2000;28(9):3301–3313.
79. et al. Infusion of very hypertonic saline to bled rats: membrane potentials and fluid shifts. J Surg Res. 1985;38(2):180–186.
80. et al. Plasma ANP during hypertonic NaCl infusion in man. Acta Physiol Scand. 1992;144(2):113–119.
81. , . Plasma expanders. An update. Am J Surg. 1988;155(3):425–434.
82. et al. Prolonged hypernatremia controls elevated intracranial pressure in head-injured pediatric patients. Crit Care Med. 2000;28(4):1136–1143.
83. et al. Use of hypertonic saline in the treatment of severe refractory posttraumatic intracranial hypertension in pediatric traumatic brain injury. Crit Care Med. 2000;28(4):1144–1151.
84. , , . Osmotic demyelination syndrome following correction of hyponatremia. N Engl J Med. 1986;314(24):1535–1542.
85. , , . Malignant cerebral edema in patients with hypertensive intracerebral hemorrhage associated with hypertonic saline infusion: a rebound phenomenon? J Neurosurg Anesthesiol. 1998;10(3):188–192.
86. et al. Cerebral extraction of oxygen and intracranial hypertension in severe, acute, pediatric brain trauma: preliminary novel management strategies. Neurosurgery. 2002;50(4):774–779; discussion 779–780.
87. et al. Brain tissue oxygen monitoring in pediatric patients with severe traumatic brain injury. J Neurosurg. 2006;105(4 suppl):281–286.
88. et al. Post-traumatic diffuse brain swelling: isolated or associated with cerebral axonal injury. Clinical course and intracranial pressure in 18 children. Childs Nerv Syst. 1987;3(4):235–238.
89. et al. Aggressive physiologic monitoring of pediatric head trauma patients with elevated intracranial pressure. Pediatr Neurosci. 1988;14(5):241–249.
90. et al. Outcome following severe head injuries in children. J Neurosurg. 1978;48(5):679–688.
91. et al. Outcome from severe head injury in children and adolescents. J Neurosurg. 1985;62(2):194–199.
92. , . High dose barbiturate therapy in neurosurgery and intensive care. Neurosurgery. 1984;15(3):427–444.
93. et al. The free radical pathology and the microcirculation in the major central nervous system disorders. Acta Physiol Scand Suppl. 1980;492:91–119.
94. et al. Alterations in cerebral blood flow, oxygen metabolism, and electrical activity produced by high dose sodium thiopental. Neurosurgery. 1980;7(6):598–603.
95. et al. The use of moderate therapeutic hypothermia for patients with severe head injuries: a preliminary report. J Neurosurg. 1993;79(3):354–362.
96. et al. Hypothermia therapy after traumatic brain injury in children. N Engl J Med. 2008;358(23):2447–2456.
97. , , . Decompressive craniotomy for acute shaken/impact baby syndrome. Pediatr Neurosurg. 1995;23(4):192–198.
98. et al. Controlled lumbar drainage in pediatric head injury. J Neurosurg. 1995;83(3):453–460.
99. et al. Nonconvulsive electrographic seizures after traumatic brain injury result in a delayed, prolonged increase in intracranial pressure and metabolic crisis. Crit Care Med. 2007;35(12):2830–2836.
100. et al. Non-convulsive seizures are common in critically ill children. Neurology. 2011;76(12):1071–1077.
101. et al. Failure of prophylactically administered phenytoin to prevent post-traumatic seizures in children. Childs Brain. 1983;10(3):185–192.
102. et al. Clinical predictors of post-traumatic seizures in children with head trauma. Ann Emerg Med. 993;22(7):1114–1118.
103. et al. Use of EEG monitoring and management of non-convulsive seizures in critically ill patients: a survey of neurologists. Neurocrit Care. 2010;12(3):382–389.
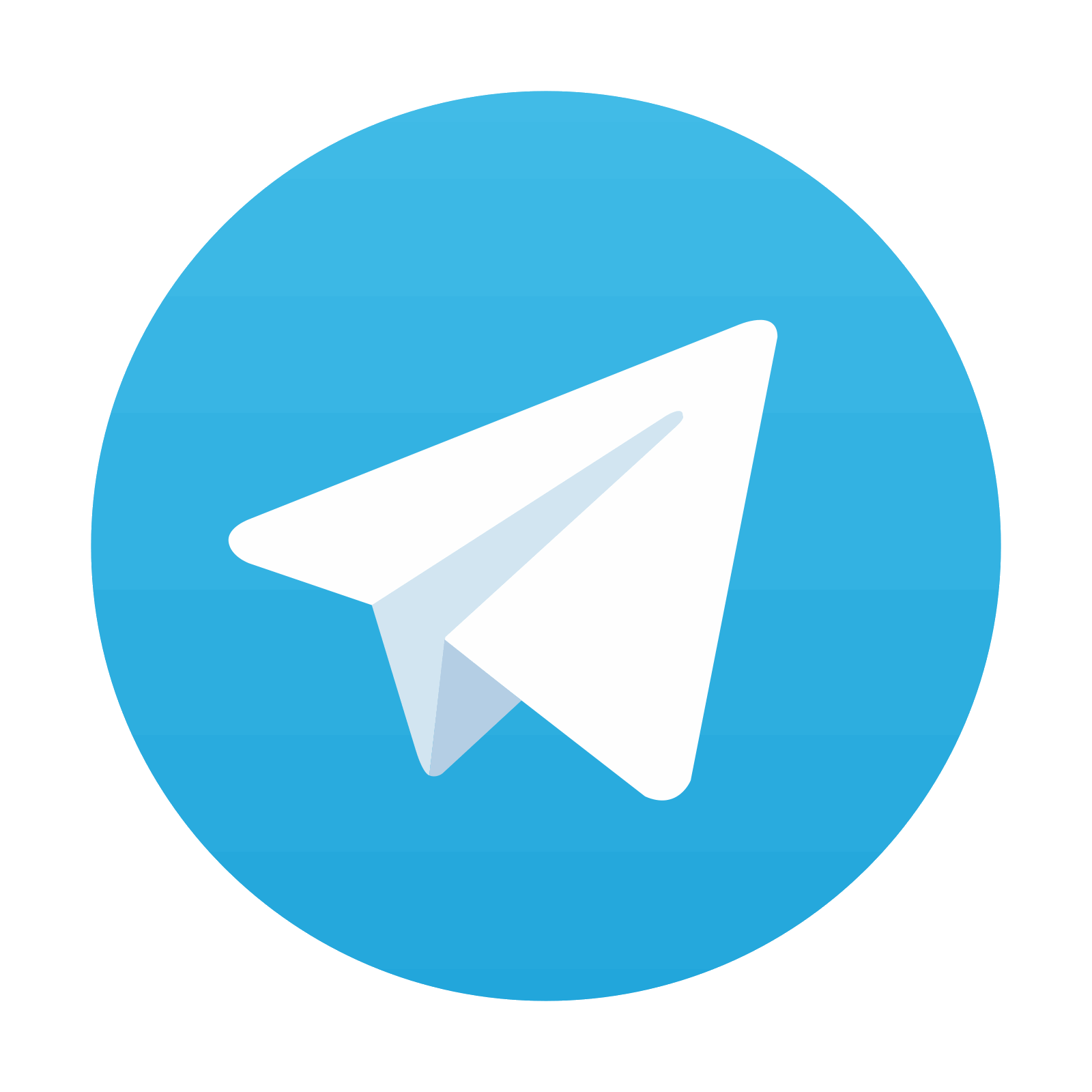
Stay updated, free articles. Join our Telegram channel
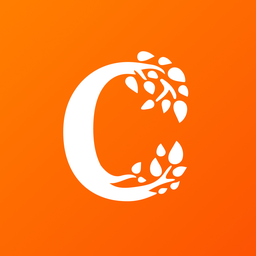
Full access? Get Clinical Tree
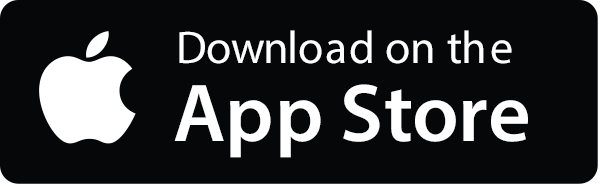
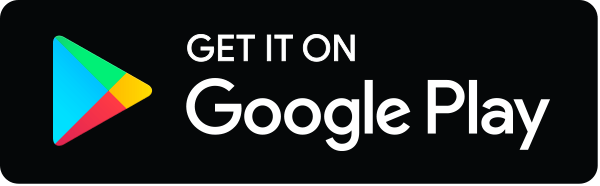